6.3: Hydrogen Bonding Interactions and Solubility
- Page ID
- 355887
\( \newcommand{\vecs}[1]{\overset { \scriptstyle \rightharpoonup} {\mathbf{#1}} } \)
\( \newcommand{\vecd}[1]{\overset{-\!-\!\rightharpoonup}{\vphantom{a}\smash {#1}}} \)
\( \newcommand{\id}{\mathrm{id}}\) \( \newcommand{\Span}{\mathrm{span}}\)
( \newcommand{\kernel}{\mathrm{null}\,}\) \( \newcommand{\range}{\mathrm{range}\,}\)
\( \newcommand{\RealPart}{\mathrm{Re}}\) \( \newcommand{\ImaginaryPart}{\mathrm{Im}}\)
\( \newcommand{\Argument}{\mathrm{Arg}}\) \( \newcommand{\norm}[1]{\| #1 \|}\)
\( \newcommand{\inner}[2]{\langle #1, #2 \rangle}\)
\( \newcommand{\Span}{\mathrm{span}}\)
\( \newcommand{\id}{\mathrm{id}}\)
\( \newcommand{\Span}{\mathrm{span}}\)
\( \newcommand{\kernel}{\mathrm{null}\,}\)
\( \newcommand{\range}{\mathrm{range}\,}\)
\( \newcommand{\RealPart}{\mathrm{Re}}\)
\( \newcommand{\ImaginaryPart}{\mathrm{Im}}\)
\( \newcommand{\Argument}{\mathrm{Arg}}\)
\( \newcommand{\norm}[1]{\| #1 \|}\)
\( \newcommand{\inner}[2]{\langle #1, #2 \rangle}\)
\( \newcommand{\Span}{\mathrm{span}}\) \( \newcommand{\AA}{\unicode[.8,0]{x212B}}\)
\( \newcommand{\vectorA}[1]{\vec{#1}} % arrow\)
\( \newcommand{\vectorAt}[1]{\vec{\text{#1}}} % arrow\)
\( \newcommand{\vectorB}[1]{\overset { \scriptstyle \rightharpoonup} {\mathbf{#1}} } \)
\( \newcommand{\vectorC}[1]{\textbf{#1}} \)
\( \newcommand{\vectorD}[1]{\overrightarrow{#1}} \)
\( \newcommand{\vectorDt}[1]{\overrightarrow{\text{#1}}} \)
\( \newcommand{\vectE}[1]{\overset{-\!-\!\rightharpoonup}{\vphantom{a}\smash{\mathbf {#1}}}} \)
\( \newcommand{\vecs}[1]{\overset { \scriptstyle \rightharpoonup} {\mathbf{#1}} } \)
\( \newcommand{\vecd}[1]{\overset{-\!-\!\rightharpoonup}{\vphantom{a}\smash {#1}}} \)
\(\newcommand{\avec}{\mathbf a}\) \(\newcommand{\bvec}{\mathbf b}\) \(\newcommand{\cvec}{\mathbf c}\) \(\newcommand{\dvec}{\mathbf d}\) \(\newcommand{\dtil}{\widetilde{\mathbf d}}\) \(\newcommand{\evec}{\mathbf e}\) \(\newcommand{\fvec}{\mathbf f}\) \(\newcommand{\nvec}{\mathbf n}\) \(\newcommand{\pvec}{\mathbf p}\) \(\newcommand{\qvec}{\mathbf q}\) \(\newcommand{\svec}{\mathbf s}\) \(\newcommand{\tvec}{\mathbf t}\) \(\newcommand{\uvec}{\mathbf u}\) \(\newcommand{\vvec}{\mathbf v}\) \(\newcommand{\wvec}{\mathbf w}\) \(\newcommand{\xvec}{\mathbf x}\) \(\newcommand{\yvec}{\mathbf y}\) \(\newcommand{\zvec}{\mathbf z}\) \(\newcommand{\rvec}{\mathbf r}\) \(\newcommand{\mvec}{\mathbf m}\) \(\newcommand{\zerovec}{\mathbf 0}\) \(\newcommand{\onevec}{\mathbf 1}\) \(\newcommand{\real}{\mathbb R}\) \(\newcommand{\twovec}[2]{\left[\begin{array}{r}#1 \\ #2 \end{array}\right]}\) \(\newcommand{\ctwovec}[2]{\left[\begin{array}{c}#1 \\ #2 \end{array}\right]}\) \(\newcommand{\threevec}[3]{\left[\begin{array}{r}#1 \\ #2 \\ #3 \end{array}\right]}\) \(\newcommand{\cthreevec}[3]{\left[\begin{array}{c}#1 \\ #2 \\ #3 \end{array}\right]}\) \(\newcommand{\fourvec}[4]{\left[\begin{array}{r}#1 \\ #2 \\ #3 \\ #4 \end{array}\right]}\) \(\newcommand{\cfourvec}[4]{\left[\begin{array}{c}#1 \\ #2 \\ #3 \\ #4 \end{array}\right]}\) \(\newcommand{\fivevec}[5]{\left[\begin{array}{r}#1 \\ #2 \\ #3 \\ #4 \\ #5 \\ \end{array}\right]}\) \(\newcommand{\cfivevec}[5]{\left[\begin{array}{c}#1 \\ #2 \\ #3 \\ #4 \\ #5 \\ \end{array}\right]}\) \(\newcommand{\mattwo}[4]{\left[\begin{array}{rr}#1 \amp #2 \\ #3 \amp #4 \\ \end{array}\right]}\) \(\newcommand{\laspan}[1]{\text{Span}\{#1\}}\) \(\newcommand{\bcal}{\cal B}\) \(\newcommand{\ccal}{\cal C}\) \(\newcommand{\scal}{\cal S}\) \(\newcommand{\wcal}{\cal W}\) \(\newcommand{\ecal}{\cal E}\) \(\newcommand{\coords}[2]{\left\{#1\right\}_{#2}}\) \(\newcommand{\gray}[1]{\color{gray}{#1}}\) \(\newcommand{\lgray}[1]{\color{lightgray}{#1}}\) \(\newcommand{\rank}{\operatorname{rank}}\) \(\newcommand{\row}{\text{Row}}\) \(\newcommand{\col}{\text{Col}}\) \(\renewcommand{\row}{\text{Row}}\) \(\newcommand{\nul}{\text{Nul}}\) \(\newcommand{\var}{\text{Var}}\) \(\newcommand{\corr}{\text{corr}}\) \(\newcommand{\len}[1]{\left|#1\right|}\) \(\newcommand{\bbar}{\overline{\bvec}}\) \(\newcommand{\bhat}{\widehat{\bvec}}\) \(\newcommand{\bperp}{\bvec^\perp}\) \(\newcommand{\xhat}{\widehat{\xvec}}\) \(\newcommand{\vhat}{\widehat{\vvec}}\) \(\newcommand{\uhat}{\widehat{\uvec}}\) \(\newcommand{\what}{\widehat{\wvec}}\) \(\newcommand{\Sighat}{\widehat{\Sigma}}\) \(\newcommand{\lt}{<}\) \(\newcommand{\gt}{>}\) \(\newcommand{\amp}{&}\) \(\definecolor{fillinmathshade}{gray}{0.9}\)How does adding hydroxyl groups increase the solubility of a hydrocarbon in water? To understand this, we must return to the two components of the free energy equation: enthalpy and entropy. For a solute to dissolve in a liquid, the solute molecules must be dispersed in that liquid. Solubility depends on how many solute molecules can be present within a volume of solution before they begin to associate preferentially with themselves rather than the solvent molecules. When the solute molecules are dispersed, whatever bonds or attractions holding the particles together in the solute are replaced by interactions between solvent and solute molecules. You might deduce that one reason diamonds are not soluble in water is that the \(\mathrm{C—C}\) bonds holding a carbon atom within a diamond are much stronger (take more energy to break) than the possible interactions between carbon atoms and water molecules. For a diamond to dissolve in water, a chemical reaction must take place in which multiple covalent bonds are broken. Based on this idea, we can conclude that the stronger the interactions between the solute particles, the less favorable it is for the solute to dissolve in water. At the same time, the stronger the interactions between solute and solvent molecules, the greater the likelihood that solubility will increase.
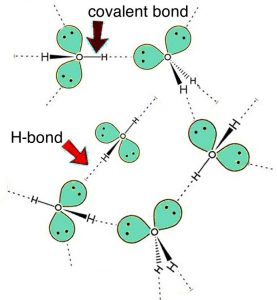
So do intermolecular interactions explain everything about solubility? Do they explain the differences between the solubility of hexane, hexanol, and hexanediol in water? Hexanediol (\(\mathrm{HO}(\mathrm{CH}_{2})_{6}\mathrm{OH}\)) is readily soluble, and if we consider its structure we can see that interactions between hexanediol molecules include hydrogen bonding (involving the two hydroxyl groups) and van der Waals interactions (LDFs and dipole-dipole). We can also approach this from a more abstract perspective. If we indicate the non-hydroxyl (\(—\mathrm{O–H}\)) part of a molecule as \(\mathrm{R}\), then an alcohol molecule can be represented as \(\mathrm{R}—\mathrm{O–H}\), and a diol can be represented as \(\mathrm{H–O}—\mathrm{R}—\mathrm{O–H}\). All alcohols have the ability to form hydrogen bonding interactions with each other as well as with water. So when an alcohol dissolves in water, the interactions between the alcohol molecules are replaced by interactions between alcohol and water molecules—an interaction similar to that between water molecules. Like water molecules, alcohols have a dipole (unequal charge distribution), with a small negative charge on the oxygen(s) and small positive charges on the hydrogen (bonded to the oxygen atoms). It makes sense that molecules with similar structures interact in similar ways. Thus, small molecular-weight alcohols can dissolve in water. But if you look again at the previous table, notice that hexanol (a 6-carbon chain with one \(—\mathrm{O–H}\) group) is much less soluble than hexanediol (a 6-carbon chain with two \(—\mathrm{O–H}\) groups—one at each end). As the non-polar carbon chain lengthens, the solubility typically decreases. However, if there are more \(—\mathrm{O–H}\) groups present, there are more possible interactions with the water. This is also why common sugars, which are really polyalcohols with large numbers of \(—\mathrm{O–H}\) groups (at least 4 or 5 per molecule), are very soluble in water. Their \(—\mathrm{O–H}\) groups form hydrogen-bonds with water molecules to form stabilizing interactions. As the length of the hydrocarbon chain increases, the non-polar hydrocarbon part of the molecule starts to become more important and the solubility decreases. This phenomenon is responsible for the “like-dissolves-like” statements that are often found in introductory chemistry books (including this one, apparently). So, do intermolecular interactions explain everything about solubility? If only things were so simple!
Entropy and Solubility: Why Don’t Oil and Water Mix?[2]
The fact that oil and water do not mix is well known. It has even become a common metaphor for other things that do not mix (people, faiths, etc.) What is not quite so well known is, why? Oil is a generic name for a group of compounds, many of which are hydrocarbons or contain hydrocarbon-like regions. Oils are – well – oily, they are slippery and (at the risk of sounding tedious) unable to mix with water. The molecules in olive oil or corn oil typically have a long hydrocarbon chain of about 16–18 carbons. These molecules often have polar groups called esters (groups of atoms that contain \(\mathrm{C—O}\) bonds) at one end.[3] Once you get more than six carbons in the chain, these groups do not greatly influence solubility in water, just as the single \(\mathrm{O–H}\) groups in most alcohols do not greatly influence solubility. So, oily molecules are primarily non-polar and interact with one another as well as with other molecules (including water molecules), primarily through London dispersion forces (LDFs). When oil molecules are dispersed in water, their interactions with water molecules include both LDFs and interactions between the water dipole and an induced dipole on the oil molecules. Such dipole–induced dipole interactions are common and can be significant. If we were to estimate the enthalpy change associated with dispersing oily molecules in water, we would discover \(\Delta \mathrm{H}\) is approximately zero for many systems. This means that the energy required to separate the molecules in the solvent and solute is about equal to the energy released when the new solvent–solute interactions are formed.
Remember that the entropy change associated with simply mixing molecules is positive. So, if the enthalpy change associated with mixing oils and water is approximately zero, and the entropy of mixing is usually positive, why then do oil and water not mix? It appears that the only possibility left is that the change in entropy associated with dissolving oil molecules in water must be negative (thus making \(\Delta \mathrm{G}\) positive.) Moreover, if we disperse oil molecules throughout an aqueous solution, the mixed system spontaneously separates (unmixes). This seems to be a process that involves work. What force drives this work?
Rest assured, there is a non-mystical explanation but it requires thinking at both the molecular and the systems level. When hydrocarbon molecules are dispersed in water, the water molecules rearrange to maximize the number of \(\mathrm{H}\)-bonds they make with one another. They form a cage-like structure around each hydrocarbon molecule. This cage of water molecules around each hydrocarbon molecule is a more ordered arrangement than that found in pure water, particularly when we count up and add together all of the individual cages! It is rather like the arrangement of water molecules in ice, although restricted to regions around the hydrocarbon molecule. This more ordered arrangement results in a decrease in entropy. The more oil molecules disperse in the water, the larger the decrease in entropy. On the other hand, when the oil molecules clump together, the area of “ordered water” is reduced; fewer water molecules are affected. Therefore, there is an increase in entropy associated with the clumping of oil molecules—a totally counterintuitive idea! This increase in entropy leads to a negative value for \(-\mathrm{T} \Delta \mathrm{S}\), because of the negative sign. Therefore, in the absence of any other factor the system moves to minimize the interactions between oil and water molecules, which leads to the formation of separate oil and water phases. Depending on the relative densities of the substances, the oily phase can be either above or below the water phase. This entropy-driven separation of oil and water molecules is commonly referred to as the hydrophobic effect. Of course, oil molecules are not afraid (phobic) of water, and they do not repel water molecules. Recall that all molecules will attract each other via London dispersion forces (unless they have a permanent and similar electrical charge).
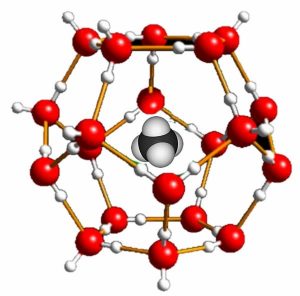
The insolubility of oil in water is controlled primarily by changes in entropy, so it is directly influenced by the temperature of the system. At low temperatures, it is possible to stabilize mixtures of water and hydrocarbons. In such mixtures, which are known as clathrates, the hydrocarbon molecules are surrounded by stable cages of water molecules (ice)(\(\rightarrow\)). Recall that ice has relatively large open spaces within its crystal structure. The hydrocarbon molecules fit within these holes, making it possible to predict the maximum size of the hydrocarbon molecules that can form clathrates. For example, some oceanic bacteria generate \(\mathrm{CH}_{4}\) (methane), which is then dissolved in the cold water to form methane clathrates. Scientists estimate that between two and ten times the current amount of conventional natural gas resources are present as methane clathrates.[4]
Solubility of Ionic Compounds: Salts
Polar compounds tend to dissolve in water, and we can extend that generality to the most polar compounds of all—ionic compounds. Table salt, or sodium chloride (\(\mathrm{NaCl}\)), the most common ionic compound, is soluble in water (\(360 \mathrm{~g/L}\)). Recall that \(\mathrm{NaCl}\) is a salt crystal composed not of discrete \(\mathrm{NaCl}\) molecules, but rather of an extended array of \(\mathrm{Na}^{+}\) and \(\mathrm{Cl}^{-}\) ions bound together in three dimensions through electrostatic interactions. When \(\mathrm{NaCl}\) dissolves in water, the electrostatic interactions within the crystal must be broken. By contrast, when molecular compounds dissolve in water, it is the intermolecular forces between separate molecules that are disrupted. One might imagine that the breaking of ionic interactions would require a very high-energy input (we have already seen that diamonds do not dissolve in water because actual covalent bonds have to be broken). That would be true if all we considered was the energy required to break the ionic interactions, as indicated by the fact that \(\mathrm{NaCl}\) melts at \(801 { }^{\circ}\mathrm{C}\) and boils at \(1413 { }^{\circ}\mathrm{C}\). But we know that substances like \(\mathrm{NaCl}\) dissolve readily in water, so clearly there is something else going on. The trick is to consider the whole system when \(\mathrm{NaCl}\) dissolves, just like we did for molecular species. We need to consider the interactions that are broken and those that are formed. These changes in interactions are reflected in the \(\Delta \mathrm{H}\) term (from \(\Delta \mathrm{G} = \Delta \mathrm{H} – \mathrm{T} \Delta \mathrm{S}).
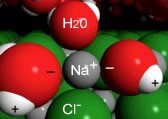
When a crystal of \(\mathrm{NaCl}\) comes into contact with water, the water molecules interact with the \(\mathrm{Na}^{+}\) and \(\mathrm{Cl}^{-}\) ions on the crystal’s surface, as shown in the figure. The positive ends of water molecules (the hydrogens) interact with the chloride ions, while the negative end of the water molecules (the oxygen) interacts with the sodium ions. So the ion on the surface of the solid interacts with water molecules from the solution; these water molecules form a dynamic cluster around the ion. Thermal motion (which reflects the kinetic energy of the molecules, that is the motion driven by collisions with other molecules in the system) then moves the ion and its water shell into solution.[5] The water shell is highly dynamic—molecules are entering and leaving it. The ion–dipole interaction between ions and water molecules can be very strongly stabilizing (\(- \Delta \mathrm{H}\)). The process by which solvent molecules interact with and stabilize solute molecules in solution is called solvation. When water is the solvent, the process is known as hydration.
Questions
Questions to Answer
- Draw a molecular-level picture of a solution of \(\mathrm{NaCl}\). Show all the kinds of particles and interactions present in the solution.
- When we calculate and measure thermodynamic quantities (such as \(\Delta \mathrm{H}\), \(\Delta \mathrm{S}\) and \(\Delta \mathrm{G}\)), why is it important to specify the system and the surroundings?
- When a substance dissolves in water, what is the system and what are the surroundings? Why? What criteria would you use to specify the system and surroundings?
- For a solution made from \(\mathrm{NaCl}\) and water, what interactions must be overcome as the \(\mathrm{NaCl}\) goes into solution? What new interactions are formed in the solution?
- If the temperature goes up when the solution is formed, what can we conclude about the relative strengths of the interactions that are broken and those that are formed? What can we conclude if the temperature goes down?
- When you measure the temperature of a solution, are you measuring the system or the surroundings?
Questions to Ponder
- Why is the water shell around an ion not stable?
- What are the boundaries of a biological system?