4.1: 3D and 2D Representations
- Page ID
- 355279
\( \newcommand{\vecs}[1]{\overset { \scriptstyle \rightharpoonup} {\mathbf{#1}} } \)
\( \newcommand{\vecd}[1]{\overset{-\!-\!\rightharpoonup}{\vphantom{a}\smash {#1}}} \)
\( \newcommand{\id}{\mathrm{id}}\) \( \newcommand{\Span}{\mathrm{span}}\)
( \newcommand{\kernel}{\mathrm{null}\,}\) \( \newcommand{\range}{\mathrm{range}\,}\)
\( \newcommand{\RealPart}{\mathrm{Re}}\) \( \newcommand{\ImaginaryPart}{\mathrm{Im}}\)
\( \newcommand{\Argument}{\mathrm{Arg}}\) \( \newcommand{\norm}[1]{\| #1 \|}\)
\( \newcommand{\inner}[2]{\langle #1, #2 \rangle}\)
\( \newcommand{\Span}{\mathrm{span}}\)
\( \newcommand{\id}{\mathrm{id}}\)
\( \newcommand{\Span}{\mathrm{span}}\)
\( \newcommand{\kernel}{\mathrm{null}\,}\)
\( \newcommand{\range}{\mathrm{range}\,}\)
\( \newcommand{\RealPart}{\mathrm{Re}}\)
\( \newcommand{\ImaginaryPart}{\mathrm{Im}}\)
\( \newcommand{\Argument}{\mathrm{Arg}}\)
\( \newcommand{\norm}[1]{\| #1 \|}\)
\( \newcommand{\inner}[2]{\langle #1, #2 \rangle}\)
\( \newcommand{\Span}{\mathrm{span}}\) \( \newcommand{\AA}{\unicode[.8,0]{x212B}}\)
\( \newcommand{\vectorA}[1]{\vec{#1}} % arrow\)
\( \newcommand{\vectorAt}[1]{\vec{\text{#1}}} % arrow\)
\( \newcommand{\vectorB}[1]{\overset { \scriptstyle \rightharpoonup} {\mathbf{#1}} } \)
\( \newcommand{\vectorC}[1]{\textbf{#1}} \)
\( \newcommand{\vectorD}[1]{\overrightarrow{#1}} \)
\( \newcommand{\vectorDt}[1]{\overrightarrow{\text{#1}}} \)
\( \newcommand{\vectE}[1]{\overset{-\!-\!\rightharpoonup}{\vphantom{a}\smash{\mathbf {#1}}}} \)
\( \newcommand{\vecs}[1]{\overset { \scriptstyle \rightharpoonup} {\mathbf{#1}} } \)
\( \newcommand{\vecd}[1]{\overset{-\!-\!\rightharpoonup}{\vphantom{a}\smash {#1}}} \)
\(\newcommand{\avec}{\mathbf a}\) \(\newcommand{\bvec}{\mathbf b}\) \(\newcommand{\cvec}{\mathbf c}\) \(\newcommand{\dvec}{\mathbf d}\) \(\newcommand{\dtil}{\widetilde{\mathbf d}}\) \(\newcommand{\evec}{\mathbf e}\) \(\newcommand{\fvec}{\mathbf f}\) \(\newcommand{\nvec}{\mathbf n}\) \(\newcommand{\pvec}{\mathbf p}\) \(\newcommand{\qvec}{\mathbf q}\) \(\newcommand{\svec}{\mathbf s}\) \(\newcommand{\tvec}{\mathbf t}\) \(\newcommand{\uvec}{\mathbf u}\) \(\newcommand{\vvec}{\mathbf v}\) \(\newcommand{\wvec}{\mathbf w}\) \(\newcommand{\xvec}{\mathbf x}\) \(\newcommand{\yvec}{\mathbf y}\) \(\newcommand{\zvec}{\mathbf z}\) \(\newcommand{\rvec}{\mathbf r}\) \(\newcommand{\mvec}{\mathbf m}\) \(\newcommand{\zerovec}{\mathbf 0}\) \(\newcommand{\onevec}{\mathbf 1}\) \(\newcommand{\real}{\mathbb R}\) \(\newcommand{\twovec}[2]{\left[\begin{array}{r}#1 \\ #2 \end{array}\right]}\) \(\newcommand{\ctwovec}[2]{\left[\begin{array}{c}#1 \\ #2 \end{array}\right]}\) \(\newcommand{\threevec}[3]{\left[\begin{array}{r}#1 \\ #2 \\ #3 \end{array}\right]}\) \(\newcommand{\cthreevec}[3]{\left[\begin{array}{c}#1 \\ #2 \\ #3 \end{array}\right]}\) \(\newcommand{\fourvec}[4]{\left[\begin{array}{r}#1 \\ #2 \\ #3 \\ #4 \end{array}\right]}\) \(\newcommand{\cfourvec}[4]{\left[\begin{array}{c}#1 \\ #2 \\ #3 \\ #4 \end{array}\right]}\) \(\newcommand{\fivevec}[5]{\left[\begin{array}{r}#1 \\ #2 \\ #3 \\ #4 \\ #5 \\ \end{array}\right]}\) \(\newcommand{\cfivevec}[5]{\left[\begin{array}{c}#1 \\ #2 \\ #3 \\ #4 \\ #5 \\ \end{array}\right]}\) \(\newcommand{\mattwo}[4]{\left[\begin{array}{rr}#1 \amp #2 \\ #3 \amp #4 \\ \end{array}\right]}\) \(\newcommand{\laspan}[1]{\text{Span}\{#1\}}\) \(\newcommand{\bcal}{\cal B}\) \(\newcommand{\ccal}{\cal C}\) \(\newcommand{\scal}{\cal S}\) \(\newcommand{\wcal}{\cal W}\) \(\newcommand{\ecal}{\cal E}\) \(\newcommand{\coords}[2]{\left\{#1\right\}_{#2}}\) \(\newcommand{\gray}[1]{\color{gray}{#1}}\) \(\newcommand{\lgray}[1]{\color{lightgray}{#1}}\) \(\newcommand{\rank}{\operatorname{rank}}\) \(\newcommand{\row}{\text{Row}}\) \(\newcommand{\col}{\text{Col}}\) \(\renewcommand{\row}{\text{Row}}\) \(\newcommand{\nul}{\text{Nul}}\) \(\newcommand{\var}{\text{Var}}\) \(\newcommand{\corr}{\text{corr}}\) \(\newcommand{\len}[1]{\left|#1\right|}\) \(\newcommand{\bbar}{\overline{\bvec}}\) \(\newcommand{\bhat}{\widehat{\bvec}}\) \(\newcommand{\bperp}{\bvec^\perp}\) \(\newcommand{\xhat}{\widehat{\xvec}}\) \(\newcommand{\vhat}{\widehat{\vvec}}\) \(\newcommand{\uhat}{\widehat{\uvec}}\) \(\newcommand{\what}{\widehat{\wvec}}\) \(\newcommand{\Sighat}{\widehat{\Sigma}}\) \(\newcommand{\lt}{<}\) \(\newcommand{\gt}{>}\) \(\newcommand{\amp}{&}\) \(\definecolor{fillinmathshade}{gray}{0.9}\)To extend our discussion to the wider world of what we might call heterogenous molecules, that is, molecules made up of atoms of more than one element, we will begin with carbon. Why carbon? Well, here are some reasons. Carbon is the fourth most abundant element in the universe (\(\sim 3,032\) atoms per million), after hydrogen (\(\sim 705,700\) atoms per million), helium (\(\sim 275,200\) atoms per million), and oxygen (\(\sim 5,920\) atoms per million). Carbon is distinguished from most other elements in its ability to form a vast array of diverse compounds by bonding with itself and other elements with bonds that are not too strong and not too weak. Under the conditions that persist on the surface of the Earth carbon compounds are stable enough to hang around but not stable enough to persist forever, so they are not dead ends. Carbon is a key building block of the major molecules of life: proteins, nucleic acids, lipids, and carbohydrates. We are carbon-based life forms! Carbon compounds are also used in a wide range of synthetic materials, such as pharmaceuticals, polymers, and high-tech materials; we also consume a lot of carbon compounds by burning them for fuel.
Carbon: Always Tetravalent and Often Tetrahedral
Atoms combine in many different ways. We have already seen an example of how a covalent bond can form between two hydrogen atoms producing molecular (\(\mathrm{H}_{2}\)) as opposed to the atomic form of hydrogen. Similarly atoms of carbon can be linked together in various ways to form diamond, graphite, and graphene (see Chapter \(3\)). Now we move on to molecules involving atoms of carbon and other elements. In keeping with our ongoing attempt to keep things simple (or better put, as simple as possible), let us start by examining the types of molecules that can be formed by combining carbon with hydrogen. There are many such molecules, and collectively they are known as hydrocarbons. The simplest such compound is methane \(\mathrm{CH}_{4}\), a major component of natural gas. As in all its compounds and its elemental forms, carbon is tetravalent, which means that it always forms four bonds. We will now consider in greater detail why this is so, what forms the bonds can take, and what are the consequences of this fact. In this discussion, we will be building on the ideas introduced when we talked about diamond, graphite, and graphene.
To answer these questions we need to return to the ideas (introduced in Chapter \(2\)) about the quantization of electron energy levels. Carbon has a total of six electrons, two of which are in a filled (\(1\mathrm{s}\)) quantum shell, and four valence electrons; it is these valence electrons that can take part in bonding. Remember that the formation of a bond always lowers the energy of a system. It therefore makes sense that a carbon atom would form as many bonds as possible, resulting in the most stable possible molecular species.
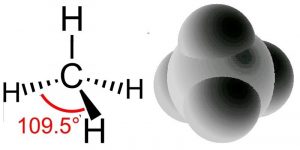
What happens if we combine hydrogen with carbon? Do we get a compound with properties intermediate between the two? Absolutely not, as you might have expected when considering the differences between diamonds and graphite. As previously we use the hybridization model to explain the behaviors we observe. We begin with what we know: in methane the carbon atoms make four bonds, one to each of four hydrogen atoms. We also know, from experiment, that the shape of the methane molecule is tetrahedral; there is a carbon at the center and the four \(\mathrm{C-H}\) bonds pointing towards the corners of a four-sided figure. Since each \(\mathrm{C-H}\) bond is formed from bonding orbitals we can use the model for bonding where these four bonding orbitals arise from the “hybridization” of the pre-existing \(2\mathrm{s}\) and three \(2\mathrm{p}\) atomic orbitals. The electrons in the \(1\mathrm{s}\) orbital are not used because the amount of energy needed to use those electrons is greater than the energy that would be released upon bond formation (they are held tightly to the nucleus by the electromagnetic force). It turns out to be a general rule that electrons in the core of the atom—in filled shells—tend not to take part in bonding. This means we need only consider the valence electrons when thinking about bonding.
The hybridization of the \(2\mathrm{s}\) and the three \(2\mathrm{p}\) orbitals results in four \(\mathrm{sp}^{3}\) molecular orbitals, each of which can interact with the \(\mathrm{H}\) atom’s \(1\mathrm{s}\) orbital to form a bond. When a bonding orbital is formed it contains two electrons. Because carbon has four valence electrons and each of the four hydrogens has one electron the result is a total of eight electrons distributed in four bonding orbitals.
Recall that we say the hybridization of carbon is \(\mathrm{sp}^{3}\) and the arrangement of the bonds is tetrahedral, which means the angle between orbitals (and the C–H bonds) is 109.5º. Another way to say this is that the \(\mathrm{H-C-H}\) bond angle is \(109.5^{\circ}\). We can predict that this will be the case based on theoretical calculations; these have been confirmed by experimental observations. But why should this be true? How many different arrangements are there for four hydrogens bonded to a single carbon? Why aren’t the hydrogens all arranged in a single plane (around a central \(\mathrm{C}\) with \(90^{\circ}\) bond angles) rather than in the tetrahedral arrangement? The planar arrangement, which is known as a square planar geometry, is actually possible and is sometimes observed under some special conditions, usually in molecules involving transition metals as we will see later). The square planar arrangement is not as stable as the tetrahedral arrangement for carbon because each \(\mathrm{C-H}\) bond can be considered as a region of high electron (negative charge) density. Given that like repels like, each bond repels the others and moves as far away from the other bonds as possible. The optimum bond angle turns out to be \(109.5^{\circ}\) away from each of their neighbors. At that point, if they moved away from one orbital they would move closer to another. You may want to convince yourself of this geometric fact by using a marshmallow, toothpicks, and gumdrops! This principle goes by the unwieldy name of valence shell electron pair repulsion (VSEPR) and can be used to predict (once you get the hang of it) the three-dimensional (3D) structure of simple molecules—assuming that you know how the atoms within a molecule are connected. For example, using VSEPR logic, you should be able to present a compelling argument for why the \(\mathrm{C-H}\) bonds in methane do not adopt a square planar orientation, as well as the general shape of many other types of molecules. You can even go further, in methane all four atoms attached to the central carbon are the same but what if they are different? You should be able to make plausible predictions about how bond angles would change if one of the attached groups is larger than the others – how would that influence bond angles?
One problem for many people is that 3D visualization of molecular structures is not easy. It is particularly tricky when one is called upon to translate the more or less abstract two-dimensional (2D) representations (Lewis and dot stuctures \(\downarrow\)) that you find printed on the page of a book, into a 3D model you can manipulate with your hands or in your mind. In addition, chemists (and molecular biologists) have an annoying tendency of representing complex 3D structures using various 2D representations, which can be confusing if you don’t know what you are looking at (or for). You have probably already seen some of these different structures, and we will consider a number of them below. Each provides specific kinds of information about the molecule. Note that actual 3D physical models and web activities can be very helpful in solidifying your ideas about structure.
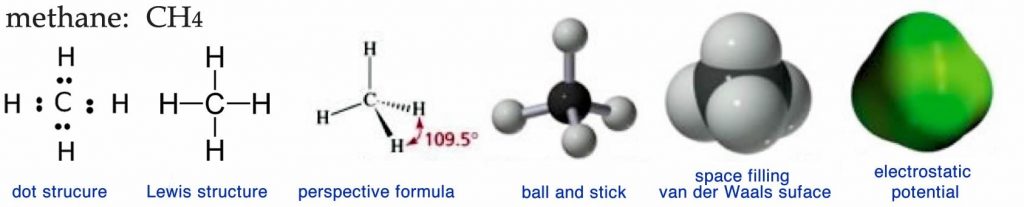
If we were able to see a methane molecule, what we observe would probably be closest to the electrostatic potential map. This visualization provides a picture of the surface of the molecule, generally color coded to represent fluctuations in electron density. Notice that there are no color fluctuations on this model of methane indicating that there are no (permanent) electron cloud distortions in the molecule—the surface of the molecule is uniformly electrically neutral. What is not so easy to discern from this representation is the fact that the methane is tetrahedral or that the central carbon atom is bonded to four hydrogen atoms, a fact that is much easier to appreciate in the other representations. The electrostatic potential representation is very useful for large biological molecules for several reasons: it is much simpler than the other kinds of models because individual atoms are not represented; it shows the molecule’s shape; and it shows where charges and partial charges are located.
The space-filling or van der Waals model gives more structural information in that the individual atoms that make up the molecule are distinguished by color (black for carbon, white for hydrogen, red for oxygen, and blue for nitrogen.) The surface of the model represents the molecule’s van der Waals radius, which is the distance where attraction turns to repulsion when two molecules approach one another. As its name implies, such models represent the space occupied by each atom.
The ball-and-stick model of methane shows the central carbon (black ball) attached to four hydrogens (white balls) by sticks that represent the bonds between the atoms. Although this model is probably the easiest to visualize, it is misleading because it could give the impression that bonds are like sticks holding the atoms together. It also does not represent either the actual volume occupied by the molecule or its electrostatic surface features. Another problem with all three of the preceding types of models is that you need a computer and specialized software (or some artistic ability) to draw them, which may not always be convenient or possible.
One strategy to address this problem is through what is known as a perspective formula. In a perspective formula the atoms are represented by their atomic symbols (for example, \(\mathrm{C}\) or \(\mathrm{H}\)) and bonds are represented by various kinds of lines. A normal line is meant to indicate a bond that is in the plane of the paper, a wedged line represents a bond that is coming out of the plane toward you (the reader), and a hatched line
represents a bond that is coming out of the plane, but away from you. This convention makes it easier to draw 3D perspective structures by hand without specialized software (or graphical talent.) We can, in fact, go one step further and draw methane without indicating its 3D structure at all. Structures that show all the bonds, atoms, and any valence electrons that are not in bonds, but do not attempt to accurately represent the 3D shape of a molecule are called Lewis structures. The Lewis structure for methane (see above) and the molecular formula \(\mathrm{CH}_{4}\) represent a chemical shorthand that can provide a huge amount of information; we will see even more extreme examples as we go on. However, to be able to understand these representations, you must already know that the methane molecule is tetrahedral and the rules that apply to the geometry of carbon bonds, because neither is shown explicitly. If you didn’t know these things, you might even be tempted to assume that methane is organized with a square planar geometry or that the hydrogens are all located to one side of the carbon atom, neither of which is true!
Why, you might ask, would one want to draw structures with so much information missing? Perhaps, like medieval alchemists, modern chemists want to keep their secrets from the average person. Perhaps they just like secret codes and mystical symbols. Or perhaps it is because these shorthand representations of molecules are just much more compact and easy to draw, particularly when we get to large molecules with lots of atoms.[1] Drawing Lewis structures is an important and useful chemistry skill and we will return to it in more detail shortly. Once you have mastered it you will be able to look at a molecular formula such as \(\mathrm{CH}_{4}\) (or \(\mathrm{C}_{5}\mathrm{H}_{12}\)) and (together with other information) be able to visualize the 3D structure of the molecule represented and predict many of the substance’s physical and chemical properties.
For example, models of the methane molecule predict that it is symmetrical. Again, this might not be entirely obvious just by looking at the structure, but if you make a model, or look at a rotatable interactive 3D model on the web you will see that it does not matter which way you look at the structure—all the \(\mathrm{C-H}\) bonds are the same, and all the bond angles are the same. A little more information (which we will discuss later on) will let you deduce that there are no permanent electron density distortions in the molecule—just as is shown by the electrostatic potential map. Together these enable you to deduce that methane molecules are attracted to one another solely through London dispersion forces (like helium atoms or hydrogen molecules). Given how weak these interactions between molecules are we might be brave enough to predict that the melting and boiling points of methane are low (melting and boiling occur at relatively low temperatures) and we would be right! Methane melts at \(91 \mathrm{~K}\) and boils at \(112 \mathrm{~K}\).[2]
Question to Answer
- Why (when present) are the four bonds formed by carbon usually arranged so that they point towards the corners of a tetrahedron?
Questions to Ponder
- If bond formation is stabilizing, why doesn’t carbon form six bonds, given that it has six electrons?
- Why doesn’t helium bond with carbon?
- What would be the consequences if carbon bonds with other atoms were very weak?
- What would be the consequences if carbon bonds with other atoms were very strong?
Building Increasingly Complex Molecules
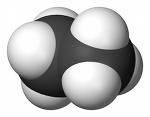
You will soon realize that it is possible to build a rather amazing number of compounds using just hydrogen and carbon. For example imagine that we remove one hydrogen from a methane molecule; this leaves us with what is known as a methyl (\(\mathrm{-CH}_{3}\)) group. We can combine two methyl groups by forming a \(\mathrm{C-C}\) bond between them (you might want to convince yourself that each carbon atom is still making four bonds with neighboring atoms). The resulting molecule is known as ethane (\(\rightarrow\)). The structure of ethane can be written in a number of ways, for example \(\mathrm{H}_{3}\mathrm{C-CH}_{3}\), \(\mathrm{CH}_{3}\mathrm{-CH}_{3}\) or \(\mathrm{C}_{2}\mathrm{H}_{6}\). As the number of atoms increases so does the number of different ways a molecule can be represented. It is for this reason that chemists have developed a number of rules that are rather strictly adhered to; these rules make it possible to unambiguously communicate the structure of a molecule to others.[3] We will not spend much time on all of these various rules but there are web activities that you can do if you want to get an introduction and to practice them. These naming conventions are controlled by the International Union of Pure and Applied Chemistry, known as IUPAC and these rules can be found in the Compendium of Chemical Terminology.[4]
The process of removing hydrogens and adding methyl groups can continue, essentially without limit, to generate a family of hydrocarbons[5] known as the alkanes; the rules that govern these molecules are simple: each hydrogen makes one and only one bond; each carbon must make four discrete bonds; and these four bonds are tetrahedral in orientation. The number of carbons is in theory unlimited and how they are linked together determines the number of hydrogens. (Can you see how two hydrocarbons with the same number of carbon atoms could have different numbers of hydrogens?)
Depending on how the carbons are connected it is possible to generate a wide variety of molecules with dramatically different shapes. For example there are cage-like, spherical, and long, string-like alkanes. Consider the four-carbon alkanes. There are butane and isobutane that have the formula \(\mathrm{C}_{4}\mathrm{H}_{10}\) as well as others with four carbons but different numbers of hydrogens, for example: cyclobutane, methylcyclopropane, and tetrahedrane. Butane has a boiling point of \(-0.5^{\circ}\mathrm{C}\), and isobutane has a boiling point of \(-11.7^{\circ}\mathrm{C}\). Why are the boiling points of butane and isobutane, which have the same atomic composition (\(\mathrm{C}_{4}\mathrm{H}_{10}\)), different? The answer lies in the fact that they have different shapes. The roughly linear carbon chain of butane has a larger surface area than isobutane, which gives it more surface area through which to interact with other molecules via London dispersion forces. This idea, that the shape of a molecule and its composition, determine the compound’s macroscopic properties is one that we will return to repeatedly.
Question to Answer
- Why are the melting and boiling points of methane higher than the melting and boiling points of \(\mathrm{H}_{2}\)?
- How many different compounds can you draw for the formula \(\mathrm{C}_{5}\mathrm{H}_{12}\)?
- What structures could you imagine for hydrocarbons containing five carbon atoms?
- Is there a generic formula for an alkane containing n carbon atoms? How does forming a ring of carbons change your formula?
- Which has the higher boiling point, a spherical or a linear alkane?
- How do boiling points and melting points change as molecular weight increases?
Question to Ponder
- Make a prediction as to the melting and boiling points of ethane, compared to methane. What assumptions are you making? How would you test whether those assumptions are valid?
- Why does the shape of a molecule influence its behavior and its macroscopic properties?