6.5: Polarity
( \newcommand{\kernel}{\mathrm{null}\,}\)
So far we have considered solutions that are made up of molecules that are either polar or non-polar or ionic species that have properties that are relatively easy to predict. Many substances, however, have more complex structures that incorporate polar, ionic, and non-polar groups. For example, many biomolecules cannot be classified as exclusively polar or non-polar, but are large enough to have distinct regions of differing polarity. They are termed amphipathic. Even though the structures of proteins such as RNA, DNA, and other biomolecules are complex, we can use the same principles involving entropic and enthalpic effects of interacting with water to understand the interactions between biomolecules, as well as within a given biomolecule. Biomolecules are very large compared to the molecules considered in most chemistry courses, and often one part of the molecule interacts with another part of the same molecule. The intramolecular[6] interactions of biological macromolecules, together with their interactions with water, are key factors in predicting their shapes.[7]
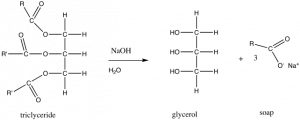
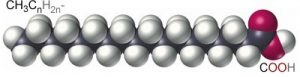
Let us begin with a relatively simple biomolecular structure. In the previous section we looked at the solubility of oils in water. Oils or fats are also known as a triglycerides. In the figure, R and R′ indicate hydrocarbon chains, which have the generic structure CH3CnH2n, shown in the figure. If you treat an oil or fat with sodium hydroxide (NaOH), the resulting chemical reaction leads to the formation of what is known as a fatty acid (in this example, oxygen atoms are maroon). A typical fatty acid has a long, non-polar hydrocarbon chain and one end that often contains both a polar and ionic group. The polar head of the molecule interacts with water with little or no increase in entropy, unlike a hydrocarbon, where the lack of H-bonding interactions with water forces a more ordered shell of water molecules around the hydrocarbon molecule, leading to a decrease in entropy. On the other hand, in water the non-polar region of the molecule creates a decrease in entropy as water molecules are organized into a type of cage around it—an unfavorable outcome in terms of ΔS, and therefore ΔG as well. So, which end of the molecule “wins”? That is do such molecules dissolve in water or not? The answer is: Both! These amphipathic molecules become arranged in such a manner that their polar groups are in contact with the water, while their non-polar regions are not. (See whether you can draw out such an arrangement, remembering to include the water molecules in your drawing.)
In fact, there are several ways to produce such an arrangement, depending in part on the amount of water in the system. A standard micelle is a spherical structure with the polar heads on the outside and the non-polar tails on the inside. It is the simplest structure that can accommodate both hydrophilic and hydrophobic groups in the same molecule. If water is limiting, it is possible to get an inverted micelle arrangement, in which polar head groups (and water) are inside and the non-polar tails point outward (as shown in the figure). Other highly organized structures can form spontaneously depending on the structure of the head group and the tail. For example, lipid molecules have multiple hydrocarbon tails and carbon ring structures called sterols. That structure creates a lipid bilayer—a polar membrane made up of two lipid molecule layers that form cellular and organellar boundaries in all organisms. It should be noted that these ordered structures are possible only because dispersing the lipid molecules in water results in a substantial decrease in the disorder of the system. In fact, many ordered structures associated with living systems, such as the structure of DNA and proteins, are the result of entropy-driven processes, yet another counterintuitive idea. This is one of the many reasons why biological systems do not violate the laws of thermodynamics and why it is scientifically plausible that life arose solely due to natural processes![8]
Questions
Questions to Answer
- If you had a compound that you suspected might form micelles:
- What structural features would you look for?
- How might you design an experiment to determine whether the compound would form micelles in water?
- What would be the experimental evidence?
- Why do you think some amphipathic molecules form spherical clusters (micelles or liposomes) whereas others form sheets (bilayers)? (Hint: consider the shape of the individual molecule itself.)
- Amphipathic molecules are often called surfactants. For example, the compounds used to disperse oil spills are surfactants. How do you think they work?
Questions to Ponder
- If membrane formation and protein folding are entropy-driven processes, does that make the origins of life seem more or less “natural” to you?
Solutions, Colloids & Emulsions
So, do micelles dissolve in water? Well, micelles are not molecules but rather supramolecular assemblies composed of many distinct molecules. A glucose solution consists of isolated glucose molecules but micelles in solution consist of larger molecular aggregates. Solutions of macromolecular solutes are called colloids. These particles can be aggregates of molecules (like micelles), atoms (nanoparticles), or larger macromolecules (proteins, nucleic acids), among others. When these particles are on the order of the wavelength of visible light, they scatter the light; smaller objects do not. This is why a salt or sugar solution is translucent, whereas a colloidal dispersion of micelles or cells is cloudy.[9] This principle also explains why soap solutions are typically cloudy—they contain particles large enough to scatter the light. When the particles in a solution maintain the structure of a solid, the end result is known as a colloid. The colloid is stable because the thermal movements of these small, solid particles are suspended. As the particles get larger, the colloid becomes unstable; the influence of gravity overcomes the effects of thermal motion and the particles settle out. Before they settle out, such unstable systems are known as suspension
But if the suspended particles are liquid, the system is known as an emulsion. For example, if we looked at a salad dressing made of oil and water under a microscope, we would see drops of oil suspended in water. Emulsions are often unstable, and over time the two liquid phases separate. This is why you have to shake up salad dressing just before using it. There are many colloids and emulsions in the world around us. Milk, for example, is an emulsion of fat globules and a colloid of protein (casein) micelles.