2.3: Pollutants with specific use
- Page ID
- 294542
2.3. Pollutants with specific use
2.3.1. Crop Protection Products
Author: Kees van Gestel
Reviewers: Steven Droge, Peter Dohmen
Leaning objectives:
You should be able to:
- describe the role of crop protection products in agriculture
- mention different types of pesticides and their different target groups.
- distinguish and mention important chemical groups of pesticides
- related to the chemistry
- related to the mode of action - describe major components included in a commercial formulation of a crop protection product beside the active substance(s).
Keywords: Insecticides, Herbicides, Fungicides, Active substances, Formulations
Introduction
Crop protection products are used in agriculture. The principle target of agriculture is the provision of food. For this purpose, agriculture aims to reduce the competition by other (non-crop) plants and the loss of crop due to herbivores or diseases. An important tool to achieve this is the use of chemicals, such as crop protection products (CPP). Accordingly, CPPs are intentionally introduced into the environment and represent one of the largest sources of xenobiotic chemicals in the environment. These chemicals are by definition effective against the target organism, often already at fairly low doses, but may also be toxic to non-target organisms including humans. The use of pesticides, also named Crop Protection Products (CPP) or often also Plant Protection Products (PPP, the latter term may be misleading for herbicides which are intended to reduce all but the crop plants), is therefore strictly regulated in most countries. The main pesticides used in the largest volumes world-wide are herbicide all s, insecticides, and fungicides. As shown in Table 1, pesticides are used against a large number of diseases and plagues.
Table 1. Classification of pesticides according to what they are supposed to control
Pesticide type |
Target |
acaricides |
against mites and spiders (incl. miticides) |
algicides |
against algae |
althelmintics (vermicides) |
against parasites |
antibiotics |
against bacteria and viruses (incl. bactericides) |
bactericides |
against bacteria |
fungicides |
against fungi |
herbicides |
against weeds |
insecticides |
against insects |
miticides |
against mites |
molluscicides |
against slugs and snails |
nematicides |
against nematodes |
plant growth regulators |
retard or accelerate the growth of plants |
repellents |
drive pests (e.g. insects, birds) away |
rodenticides |
against rodents |
Formulations
A pesticidal product usually consists of one or more active substances, that are brought onto the market in a commercial formulation (spray powder, granulate, liquid product etc.). The formulation is used to facilitate practical handling and application of the chemical, but also to enhance its effect or its safety of use. The active substance may, for instance, be a solid chemical, while application requires it to be sprayed. Or the active substance degrades fast under the influence of sunlight and therefore has to be encapsulated. One of the most used types of formulation is a concentrated emulsion, which may be sprayed directly after dilution with water. In this formulation, the active substance is dissolved in an oily matrix and a detergent is added as emulsifier to make the oil miscible with water. In this way, the active substance becomes quickly available after spraying. In so-called slow-release formulations, the active substance is encapsulated in permeable microcapsules, from which it is slowly released. Another component of a formulation can be a synergist, which increases the efficacy of the active substance, for instance by blocking enzymes that metabolize the active substance. Here an overview of main formulation constituents:
- Solvents: to ease handling and application of the active substances, they are usually dissolved. For a highly water soluble compound this solvent may just be water; however, most compounds have low water solubility and they are thus dissolved in organic solvents.
- Emulsifier, detergents, dispersants: used to provide a homogeneous mixture of the active substance in the aqueous spray solution.
- Carrier: solid formulations, such as wettable granules (WG). All wettable powder (WP) formulations often use inert materials such as clay (kaolinite) as carrier.
- Wetting agent: they help providing a homogeneous film on the plant surface.
- Adjuvant: may help to increase uptake of the active substance into the plant.
- Minor constituents:
- Antifreeze agent: to keep the formulation stable also in cold storage conditions.
- Antifoam agent: some of the formulants may result in foaming during application, which is not wanted.
- Preservative, biocide: to prevent biological degradation of the active substance or the formulants during storage.
- There are numerous additional specific additional constituents for specific purposes such as colours, detterents, stickers, etc
Four types of nomenclature are used in case of pesticides:
1. The trade name, e.g. Calypso®, which is given by the manufacturer. The same active substance is often sold under more than one different trade names (accordingly, the use of trade names only is not a sufficient description of the test substance in scientific literature).
2. The code name, which is the "common" name of the active substance. Calypso® 480 SC, for example, is a concentrated suspension containing 480 g/L of the active substance thiacloprid.
3. The chemical name of the active substance. Thiacloprid is [3-[(6-chloropyridin-3-yl)methyl]-1,3-thiazolidin-2-ylidene]cyanamide.
4. The name of the chemical group to which the active substance belongs, in case of thiacloprid: neonicotinoids.
Chemical classes
Pesticides represent quite a number of different groups of chemicals. Pesticides include inorganic chemicals (like copper used as a fungicide), organic synthetic chemicals, and biologicals (organic natural compounds). Pesticides from the same chemical group may be used against different pest organisms, like the organotin compounds (see below). Some chemicals have a broad mode of action: many soil disinfectants, such as metam-sodium, kill nematodes, fungi, soil insects and weeds. Other pesticides are more selective, like neonicotinoids acting only on insects, or very selective, like the insect-growth regulator fenoxycarb, which is used against leaf-rollers without affecting its natural enemies. Selectivity of a pesticide also indicates to what extent non-target species may be affected upon its application (side-effects). Integrated pest management (IPM) aims at an as sustainable as possible crop protection system by combining biological agents (predators of the pest organism) using chemicals having a selective mode of action. Such systems are nowadays receiving increasing interest in different agricultural crops.
Some groups of pesticides that were used or still are widely used are presented in more detail. Their modes of action are discussed in Chapter 4.
- Chlorinated hydrocarbons
Best known representative of this group is DDT (dichloro diphenyl trichloroethane; Figure 1), which was discovered in 1939 by the Swiss entomologist Paul Hermann Müller and seemed to be an ideal pesticide: it was effective, cheap and easy to produce and remained active for a long period of time. As a remedy against Malaria and other insect borne diseases, it has saved millions of human lives. However, the high persistency of DDT, its strong bioaccumulation and its effects on bird populations have triggered the search for alternatives and its ban in most Western countries. But in some developing countries, because of a lack of suitable alternatives for an effective control of malaria, DDT is still in use to kill malaria mosquitos.
Other representatives of chlorinated hydrocarbons are lindane, also called gamma-hexachlorocyclohexane (Figure 1), and the cyclodienes that include the "drins" (aldrin, dieldrin, endrin, See Section 2.1) and endosulfan (Figure 1). Because of their high persistence and bioaccumulative potential, most organochlorinated pesticides have been banned.
Volatile halogenated hydrocarbons were often used as soil disinfectant. These compounds were injected into the soil, and acted as a nematicide but also killed fungi, soil insects and weeds. An example is 1,3-dichloropropene (Figure 1).
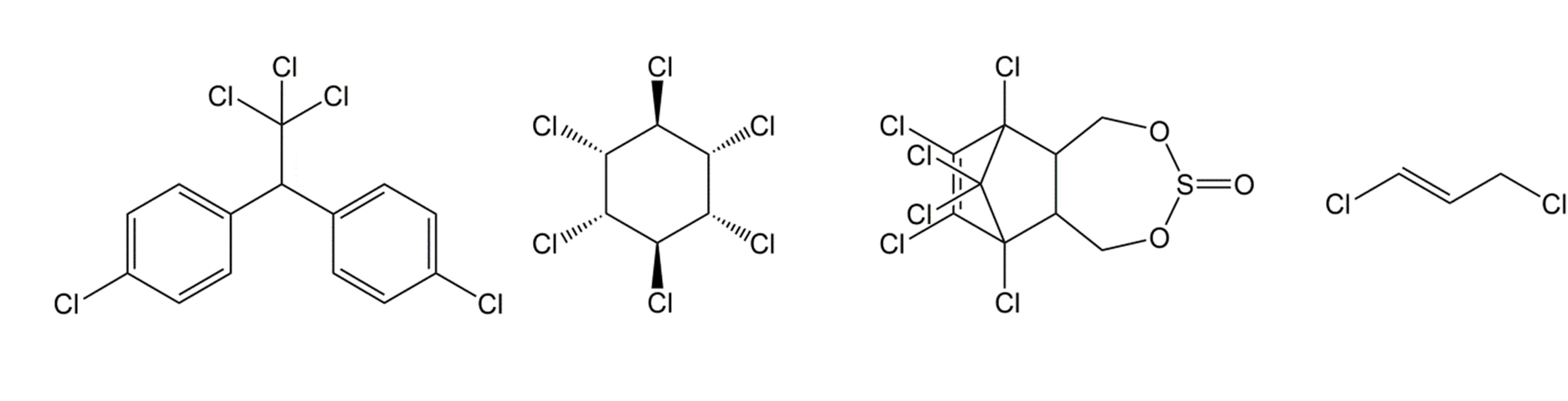
- Organophosphates
Organophosphates are esters of phosphoric acid and constitute important biological molecules such as nucleic acids (DNA) or ATP. Within the contents of pesticides this refers mainly to a group of organophosphate molecules which interfere with acetylcholinesterase. Nerve gases, produced for chemical warfare (e.g., Sarin), also belong to the organophosphates. They are much less persistent and were therefore introduced as alternatives for the chlorinated hydrocarbons. The common molecular structure of organophosphates is a tri-ester of phosphate, phosphonate, phosphorthionate, phosphorthiolate, phosphordithionate or phosphoramidate (Figure 2). With two of the three ester bonds, a methyl- or ethyl- group is bound to the P atom, while the third ester bond binds the rest group or "leaving group".
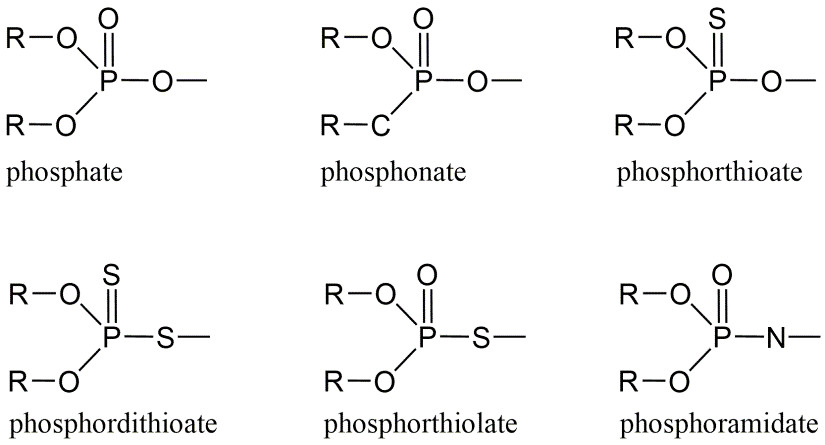
Dependent on the identity of the latter group, three sub-groups may be distinguished:
1. Aliphatic organophosphates, including malathion (Figure 3) and a number of systemic chemicals.
2. Phenyl-organophosphates, which are more stable than the aliphatic ones but also less soluble in water, like parathion (no longer allowed in Europe; Figure 3).
3. Heterocyclic organophosphates, including chemicals with an aromatic ring containing a nitrogen atom like chlorpyrifos (Figure 3).
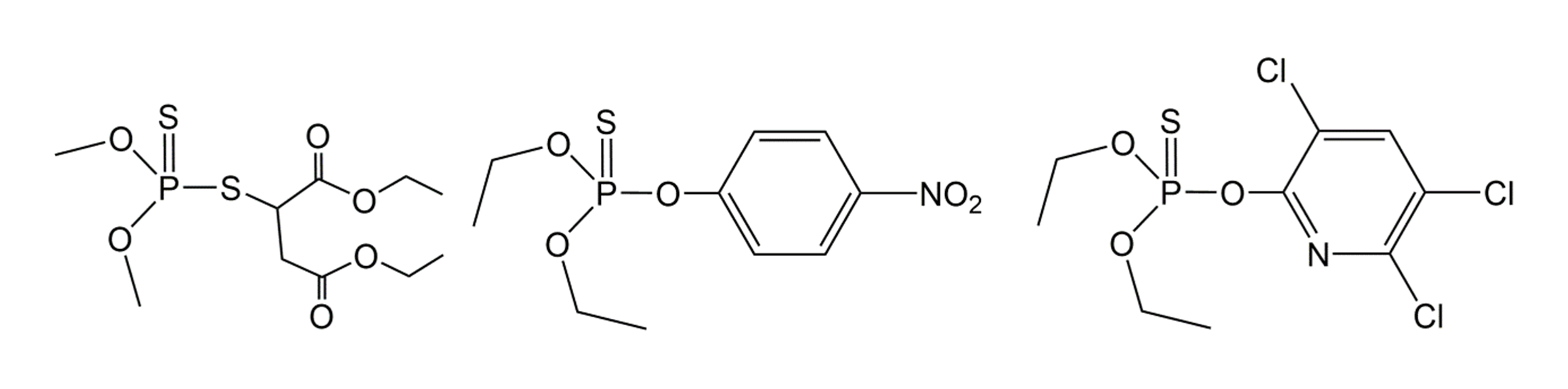
- Carbamates
Where organophosphates are derived from phosphoric acid, carbamates are derived from carbamate (Figure 4). Their mode of action is similar to that of the organophosphates. The use of older representatives of this group, like aldicarb, carbaryl, carbofuran and propoxur, is no longer allowed in Europe, but diethofencarb (Figure 4), oxamyl and methomyl are still in use.
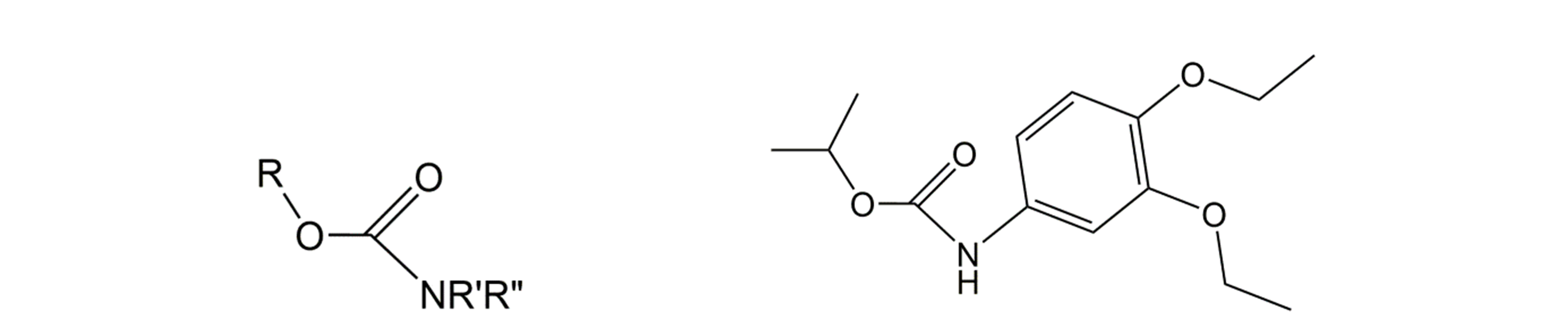
- Pyrethroids
A number of modern pesticides are derived from natural products. Pyrethroids are based on pyrethrum, a natural insecticide from flowers of the Persian ox-eyed daisy, Chrysanthemum roseum. Typical for the molecular structure of pyrethroids is the cyclopropane-carboxyl group (the triangular structure), which is connected with an aromatic group through an ester bond (Figure 5). Pyrethrum is rapidly degraded under the influence of sunlight. Synthetic pyrethroids, which are much more stable and therefore used on a large scale against many different insects, include cypermethrin (Figure 5), deltamethrin, lambda-cyhalothrin, fluvalinate and esfenvalerate.
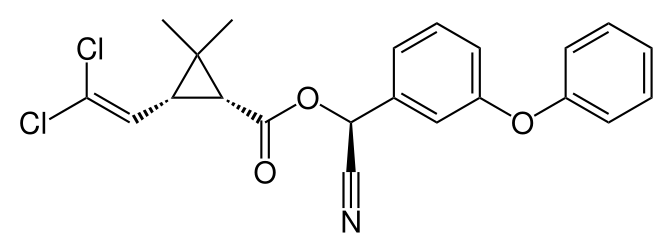
Neonicotinoids
Based on the natural compound nicotine, which acts as a natural insecticide against plant herbivores, but which was banned as an insecticide due to its high human toxicity, in the 1980s a new group of more specific insecticides has been developed, the neonicotinoids (Figure 6). Several neonicotinoids (e.g., imidacloprid, thiamethoxam) are systemic. This means that they are taken up by the plant and exert their effect from inside the plant, either on the pest organism (systemic fungicides or insecticides) or on the plant itself (systemic herbicides). The systemic neonicotinoids are widely applied as seed dressing in major crops like maize and sunflower. Other compounds are mainly used in spray applications, e.g. in fruit growing (thiacloprid, acetamiprid, etc.). Although neonicotinoids are more selective and therefore preferred over the older classes of insecticides like organophosphates, carbamates and pyrethroids, in recent years they have become under debate because of their side effects on honey bees and other pollinators.
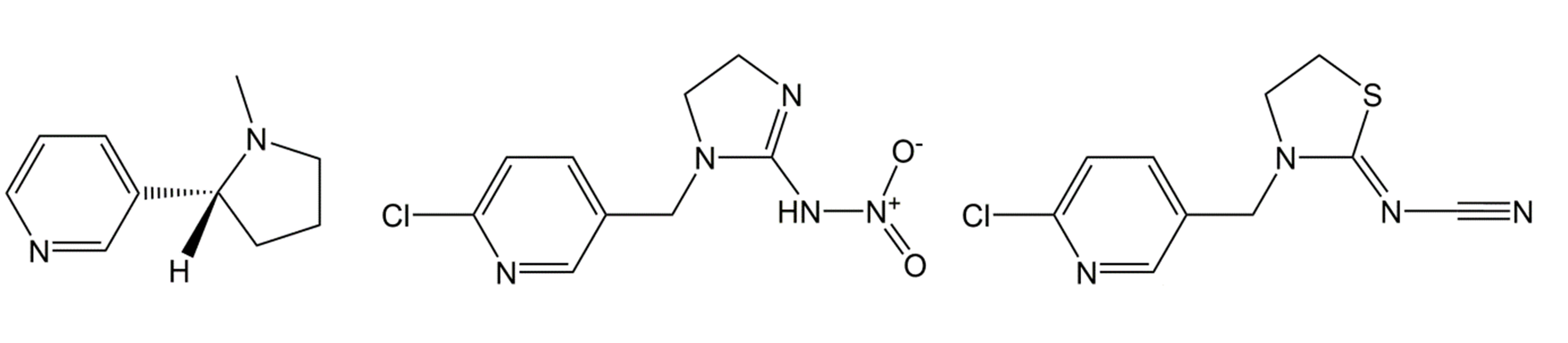
- Isothiocyanates
Isothiocyanates were used on a large scale as soil disinfectant against nematodes, fungi and weeds. The large number of chemicals with different chemical origin belonging to the isothiocyanates have in common that they form isothiocyanate in soil. A representative of this group is metam-sodium (Figure 7).
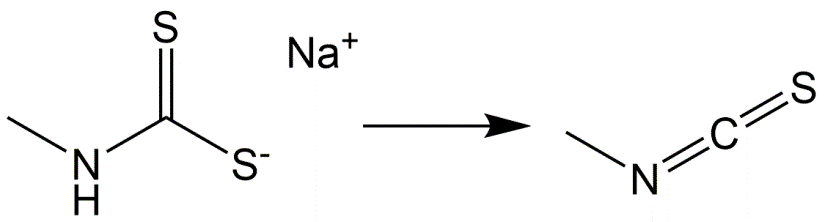
- Organotin compounds
Fentin hydroxide (Figure 8) was used as a fungicide against Phytophthora (causing potato -disease). Tributyltin compounds (TBT) were used as anti-fouling agent (algicide) on ships. TBTC (tributyltin chloride) is extremely toxic to shell-fish, such as oysters, and for this reason banned in many countries. Fenbutatin-oxide was used as an acaricide against spider mites on fruit trees (tributyltin chloride).
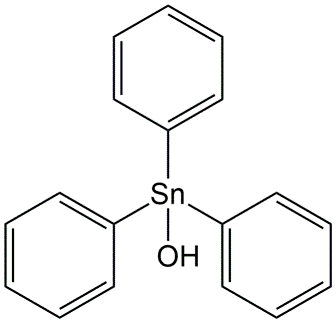
- Ryanoids
Also indicated as diamide insecticides, this group includes chemically distinct synthetic compounds such as chlorantraniliprole (Figure 9), flubendiamide, and cyantraniliprole, that act on the ryanodine receptor and are used against chewing and sucking insects.
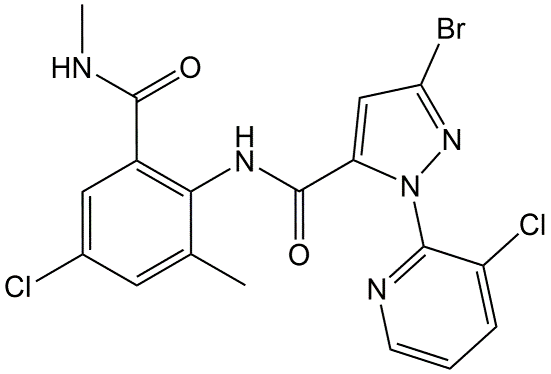
- Phenoxy acetic acids
Phenoxy acetic acids are systemic herbicides, exerting their action after uptake by the leaf and translocation throughout the plant. Especially plants with broad, horizontally oriented leaves are sensitive for these herbicides. 2,4-D (Figure 10) is the best known representative of this group.
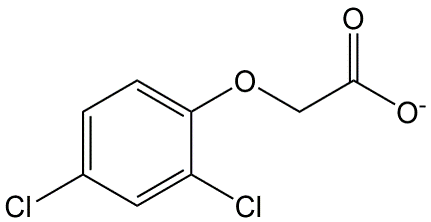
- Triazines
Triazines are heterocyclic nitrogen compounds, whose structure is characterized by an aromatic ring in which three carbon atoms have been replaced by nitrogen atoms. Triazines are usually applied to the soil before seed germination. The use of several compounds (atrazine, simazine) has been banned in Europe, while others like metribuzin and terbuthylazine (Figure 11) are still in use.
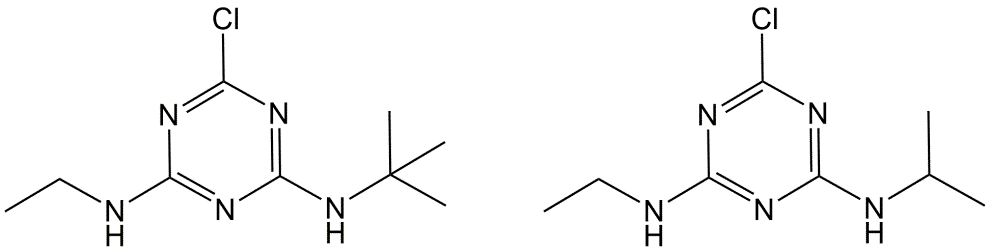
- Bipiridyls
This group contains the herbicides diquat and paraquat (Figure 12) which mainly act as contact herbicides. This means they damage the plant without being taken up. In soil, they are rapidly inactivated by strong binding to soil particles. The use of paraquat is no longer allowed in Europe, but diquat is still in use.

- Glyphosate and Glufosinate
As an alternative to the above mentioned herbicides, glyphosate and later glufosinate were developed. These are systemic broad-spectrum herbicides with a relatively simple chemical structure (Figure 13). Their low toxicity to other organisms triggered pesticide producers to introduce genetically modified crops (e.g. soybean, maize, oilseed rape, and cotton) that contain incorporated genes for resistance against these broad-spectrum herbicides. This type of resistance allows the farmer to use the herbicide without damaging the crop. For this reason, environmentalist fear an unrestricted use of these herbicides, which indeed is the case especially for glyphosate (better known under the formulation name Roundup®).
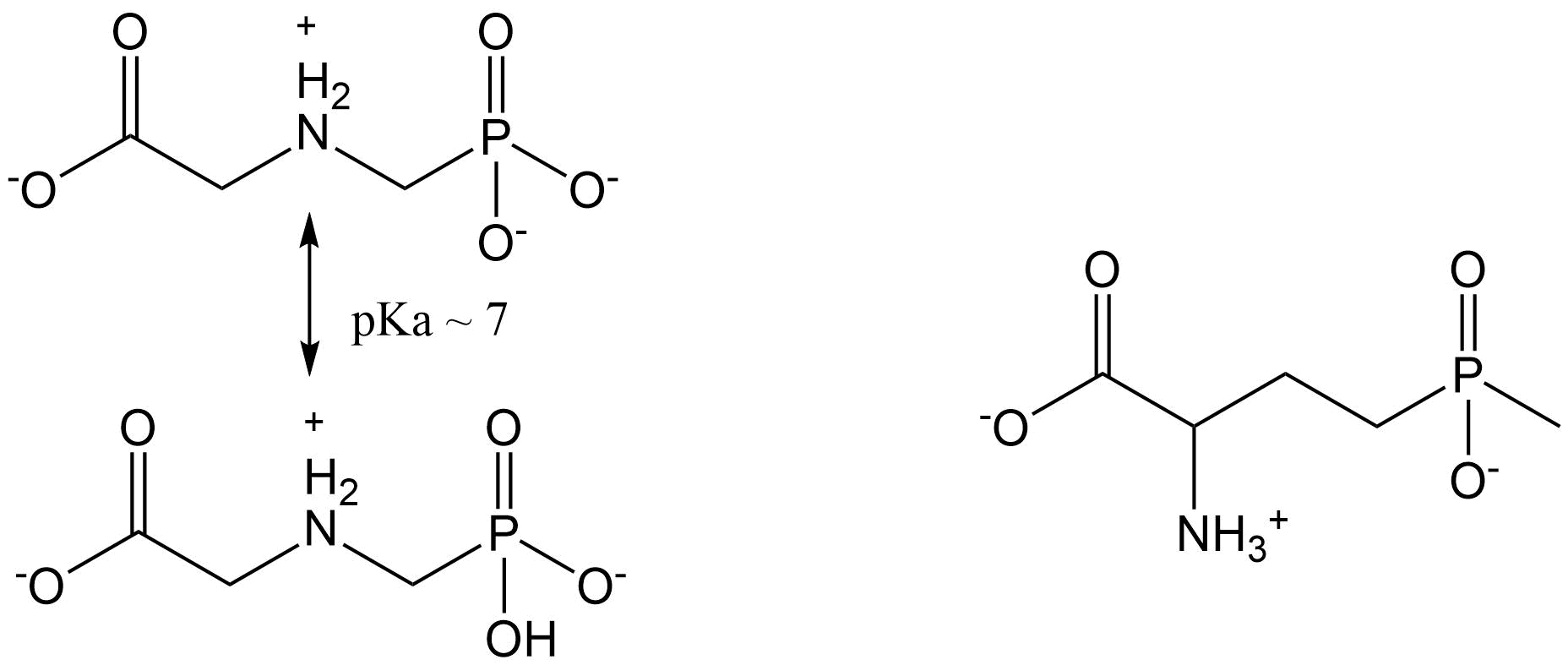
- Triazoles
Several modern fungicides are sharing a triazole group (Figure 14). These fungicides have gained importance because of problems with the resistance of fungi against other classes of fungicides. Members of this group for instance are epoxiconazole, propiconazole and tebuconazole.
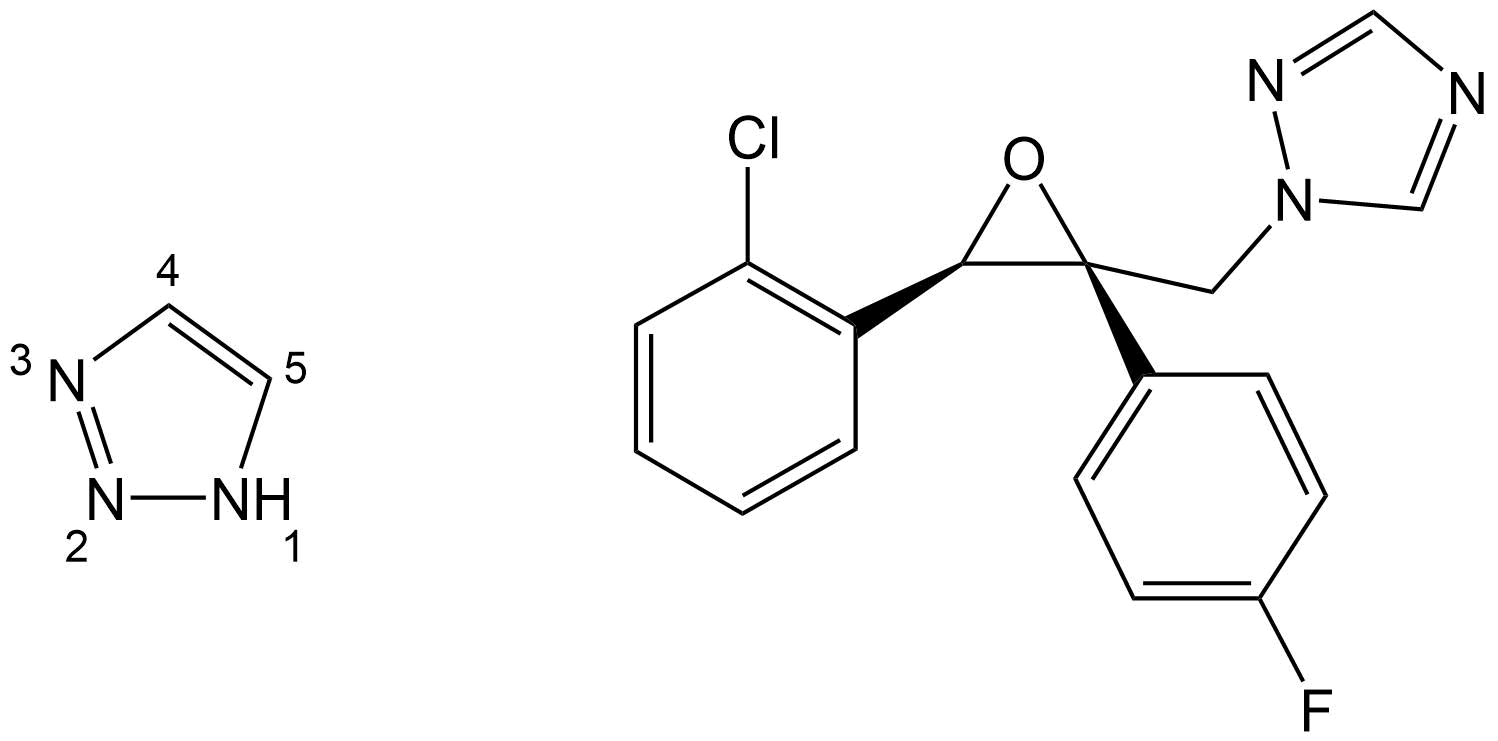
- Biological pesticides
Biological pesticides are produced in living organisms as secondary metabolites to protect themselves against predators, herbivores, parasites or competition. They can be highly effective and act at low concentrations (high toxicity), but in contrast to some synthetic pesticides they are usually sufficiently biodegradable. Compounds like pyrethrum or strobilurin are produced within the plant or within the fungus and are thus protected against photolysis or other environmental degradation. Furthermore, the living organism can produce additional quantities of the secondary metabolite on demand. When used as a pesticide applied as a spray, however, the molecule needs to be modified to enhance its stability (for example against photolysis) to remain sufficiently active over a sufficient period of time. Accordingly, synthetic derivatives of these biological molecules are often more stable, less biodegradable. Examples are the Bt insecticide, which contains an endotoxin highly toxic to insects produced by the bacterium Bacillus thuringiensis, and avermectins, complex molecules synthesized by the bacterium Streptomyces avermitilis. Avermectins act as insecticides, acaricides and have anthelminthic properties. In nature, eight different forms of avermectin have been found. Ivermectin is a slightly modified structure that is synthesized and marketed commercially. Other compounds belonging to this group are milbemectin and emamectin.
Genetically modified plants containing a gene coding for the toxin produced by the bacterium Bacillus thuringiensis (or Bt) are another example of genetic modification being applied in agriculture produce insect-resistant crops.
References:
EU Pesticides Database
Systemic pesticides are easily taken up by plants and internally distributed over all plant tissues. What do you expect regarding the water solubility of systemic pesticides?
- Very Low
- Very high
Why are systemic insecticides applied as seed dressing, so dosed into the soil, dangerous for honey bees and other pollinators?
A pesticide with an active substance that is hardly soluble in water is introduced to the market in a commercial formulation. What components should be present in the formulation to allow homogenous application of the pesticide by spraying?
Name three important groups of insecticides and mention one typical property or characteristic.
- The figures shows three chemical structures. Indicate which of these chemicals is
- An organophosphate (circle)
- A pyrethroid (triangel)
- A neonicotinoid (square)
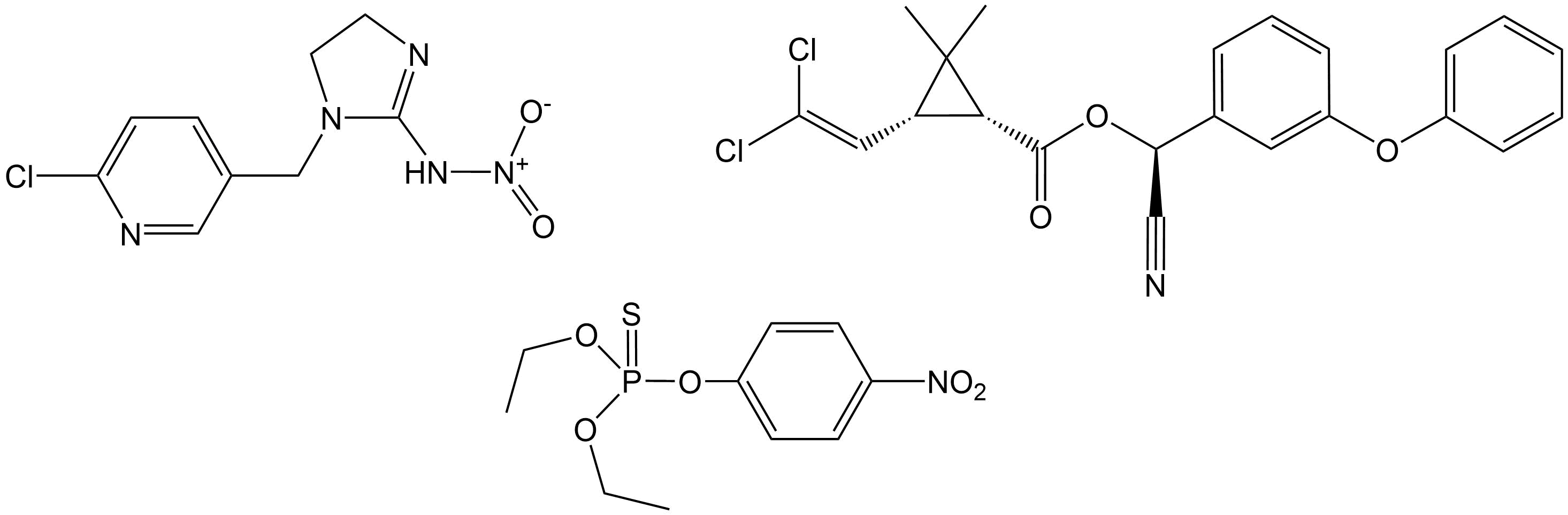
2.3.2. Biocides
Author: Thomas Wagner
Reviewers: Steven Droge, Kevin Thomas
Learning objectives:
You should be able to:
- Understand the purpose of using biocides
- Distinguish different groups of biocides
- Understand the legislation concerning the production and use of biocides
- Understand the potential impact of biocides on ecosystems
Keywords: Biocides, product types, Biocidal Product Regulation (BPR), environmental impact
Introduction
European legislation describes a biocide as 'chemical substance or microorganism intended to destroy, deter, render harmless, or exert a controlling effect on any harmful organism by chemical or biological means'. The US Environmental Protection Agency (EPA), an independent agency of the U.S. federal government to protect the environment, defines biocides as 'a diverse group of poisonous substances including preservatives, insecticides, disinfectants and pesticides used for the control of organisms that are harmful to human or animal health or that cause damage to natural or manufactured products'. The definition by the EPA includes pesticides (Chapter 2.3.1). In the scientific and non-scientific literature, the distinction between biocides, pesticides and plant protection products is often vague.
Biocides are used all around us:
- The toothpaste that you used this morning contains biocides to preserve the toothpaste
- The water that you used to flush you mouth is prepared with biocides for disinfection
- The clothes that you are wearing are impregnated with biocides to prevent smells
- The food that you ate for breakfast might have contained biocides to preserve the food
- The construction materials around you have surface coatings that contain biocides to prevent biological degradation of the material
A biocide contains an 'active substance', which is the chemical that is toxic to its target organism, and often contain 'non-active co-substances', which could help in reaching desired product parameters, such as a viscosity, pH, colour, odour or increase its handling or effectiveness. The combination of active substances and non-active substances together makes up the 'biocidal product'. An example of a well-known biocidal product is TriChlor, which contains active substance chlorine that is used to disinfect swimming pools. Because it is impractical to store chlorine gas for the treatment of swimming pools, TriChlor tablets are added to the pool water. TriChlor is trichloroisocyanuric acid (Figure 1). When dissolved in water, the Cl atoms are replaced by H atoms, forming chlorine (Cl-) and cyanuric acid (Figure 2). The free chlorine is able to disinfect the swimming pool.
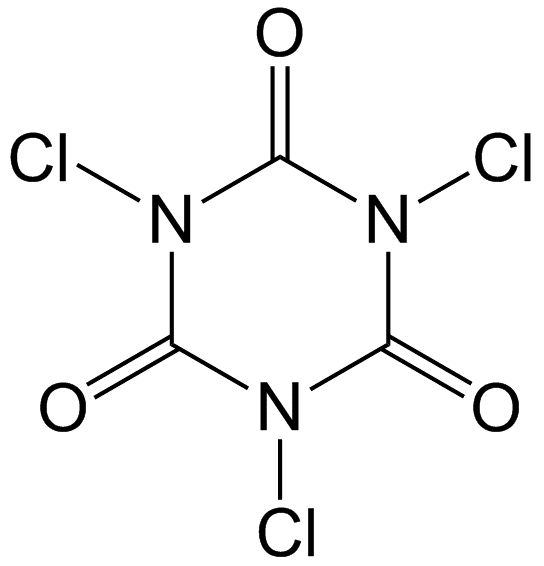
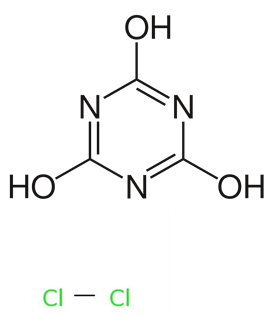
A biocidal product can also contain multiple biologically active substances to enhance its effectivity, such as AQUCAR™ 742 produced by DuPont. It contains glutaraldehyde (Figure 3) and quaternary ammonium compounds (Figure 4) that have a synergistic toxic effect on microorganisms that are present in oilfields and could form biofilms in the pipelines.
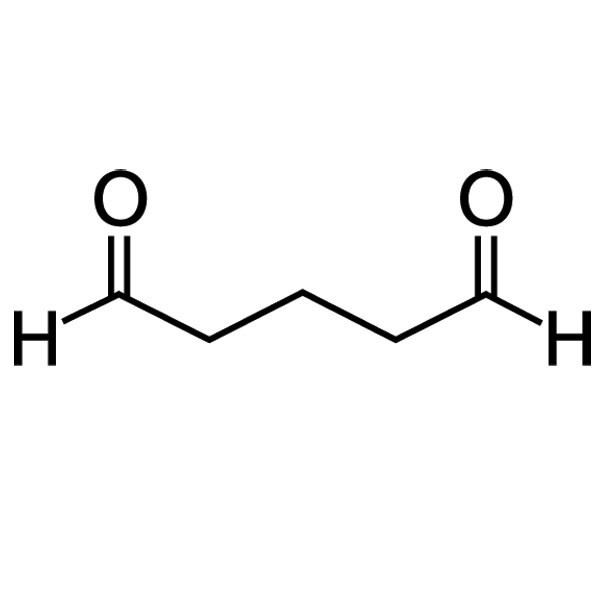
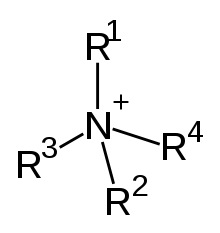
Product types
The biocidal products are classified into 22 different product-types by the European Chemicals Agency (ECHA) (Table 1). It is possible that an active substance can be classified in more than one product types.
Table 1. The classification of biocides in 22 product types (www.echa.europe.eu)
Main group 1: Disinfectants and general biocidal products |
Product type 1 - Human hygiene biocidal products |
Product type 2 - Private area and public health area disinfectants and other biocidal products |
Product-type 3 - Veterinary hygiene biocidal products |
Product type 4 - Food and feed area disinfectants |
Product-type 5 - Drinking water disinfectants |
Main group 2: Preservatives |
Product-type 6 - In-can preservatives |
Product-type 7 - Film preservatives |
Product-type 8 - Wood preservatives |
Product-type 9 - Fibre, leather, rubber and polymerised materials preservatives |
Product-type 10 - Masonry preservatives |
Product-type 11 - Preservatives for liquid-cooling and processing systems |
Product-type 12 - Slimicides |
Product-type 13 - Metalworking-fluid preservatives |
Main group 3: Pest control |
Product-type 14 - Rodenticides |
Product-type 15 - Avidicides |
Product-type 16 - Molluscicides |
Product-type 17 - Piscicides |
Product-type 18 - Insecticides, acaricides and products to control other arthropods |
Product-type 19 - Repellents and attractants |
Product-type 20 - Control of other vertebrates |
Main group 4: Other biocidal products |
Product-type 21 - Antifouling products |
Product-type 22 - Embalming and taxidermist fluids |
Legislation
In Europe, biocides are authorised for production and use by the Biocidal Products Regulation (BPR, Regulation (EU) 528/2012) of the ECHA. The BPR 'aims to improve the functioning of the biocidal product market in the EU, while ensuring a high level of protection for humans and the environment.' (echa.europe.eu/legislation). This is an alternative regulatory framework than that for the plant protection products, managed by the European Food Safety Authority (EFSA). All biocidal products go through an extensive authorisation process before they are allowed on the market. The assessment of a new active starts with the evaluation of a product by the authorities of an ECHA member state, after which the ECHA Biocidal Products Committee forms an opinion. The European Commission then makes a decision to approve or reject the new active substance based on the opinion of ECHA. This approval is granted for a maximum of 10 years and needs to be renewed after it reaches the end of the registration period. The BPR has strict criteria for new active substances, and meeting the following 'exclusion criteria' will result in the new active substance not being approved:
- Carcinogens, mutagens and reprotoxic substances categories 1A or 1B according to CLP regulation
- Endocrine disruptors
- Persistent, bioaccumulative and toxic (PBT) substances
- Very persistent and very bioaccumulative (vPvB) substances
In very special cases, new active substances will be allowed on the market when meeting this exclusion criteria, if they are important for public health and public interest and there are no alternatives available. To lower the pressure on public health and the environment, there is also a candidate list for active substances to be substituted for less harmful active substances when the old active substances meet the following criteria:
- It meets one of the exclusion criteria
- It is classified as a respiratory sensitizer
- Its toxicological reference values are significantly lower than those of the majority of approved active substances for the same product-type and use
- It meets two of the criteria to be considered as PBT
- It causes concern for human or animal health and for the environment even with very restrictive risk management measures
- It contains a significant proportion of non-active isomers or impurities
The impact of environmental release
The release of biocides in the environment can have huge consequences, since these products are designed to cause damage to living organisms. A classic example is the release of tributyltin from shipyards, harbours and on sailing routes from the antifouling paint on the hulls of ships (De Mora, 1996). Tributyltin was used in the antifouling paint from the 1950s on to prevent microorganisms from settling on the hulls of ships, which would increase the fuel costs and repair costs. However, the release of tributyltin from the paint resulted in a toxic effect on organisms at the bottom of the food chain, such as algae and invertebrates. Tributyltin then biomagnified in the food web, this way affecting larger predators, such as dolphins and sea otters. Eventually, tributyltin entered the diet of humans. The first legislation on the use of tributyltin for ships dates back to the 1980s, but it was not until the Rotterdam Convention of 2008 that the complete use of tributyltin as an active biocide in antifouling paints was banned. Biocides can also have an effect on the capability of the environment to deal with pollution. Microorganisms are responsible for cleaning polluted areas by using the pollutant as food-source. McLaughlin et al. (2016) studied the effect of the release of biocide glutaraldehyde in spilled water from hydraulic fracturing on the microbial activity and found that the microbial activity was hampered by the biocide glutaraldehyde. Hence, because of the biocide, the environment was not or slower capable to return to its original state.
References
De Mora, S.J. (1996). Tributyltin: case study of an environmental contaminant, Vol. 8, Cambridge Univ. Press
McLaughlin, M.C., Borch, T., Blotevogel, J. (2016). Spills of hydraulic fracturing chemicals on agricultural topsoil: Biodegradation, sorption and co-contaminant interactions, Environmental Science & Technology 50, 6071-6078
Explain the difference between an active substance and a biocidal product.
What is the purpose of using non-active co-substances in a biocidal product?
A ship is transporting bananas from Costa Rica to the Netherlands. Mention 5 biocide product types that could be used on this ship, and their purpose.
Mention 3 potential ways in which the environment can be damaged as the result of the (accidental) release of biocides in common daily practice.
2.3.3. Pharmaceuticals and Veterinary Pharmaceuticals
(draft)
Author: Thomas ter Laak
Reviewer: John Parsons, Steven Droge
Leaning objectives:
You should be able to:
Keywords: emission, immission, waste water treatment, disease treatment
Introduction
Pharmaceuticals are consumed by humans (human pharmaceuticals) and administered to animals (veterinary pharmaceuticals).
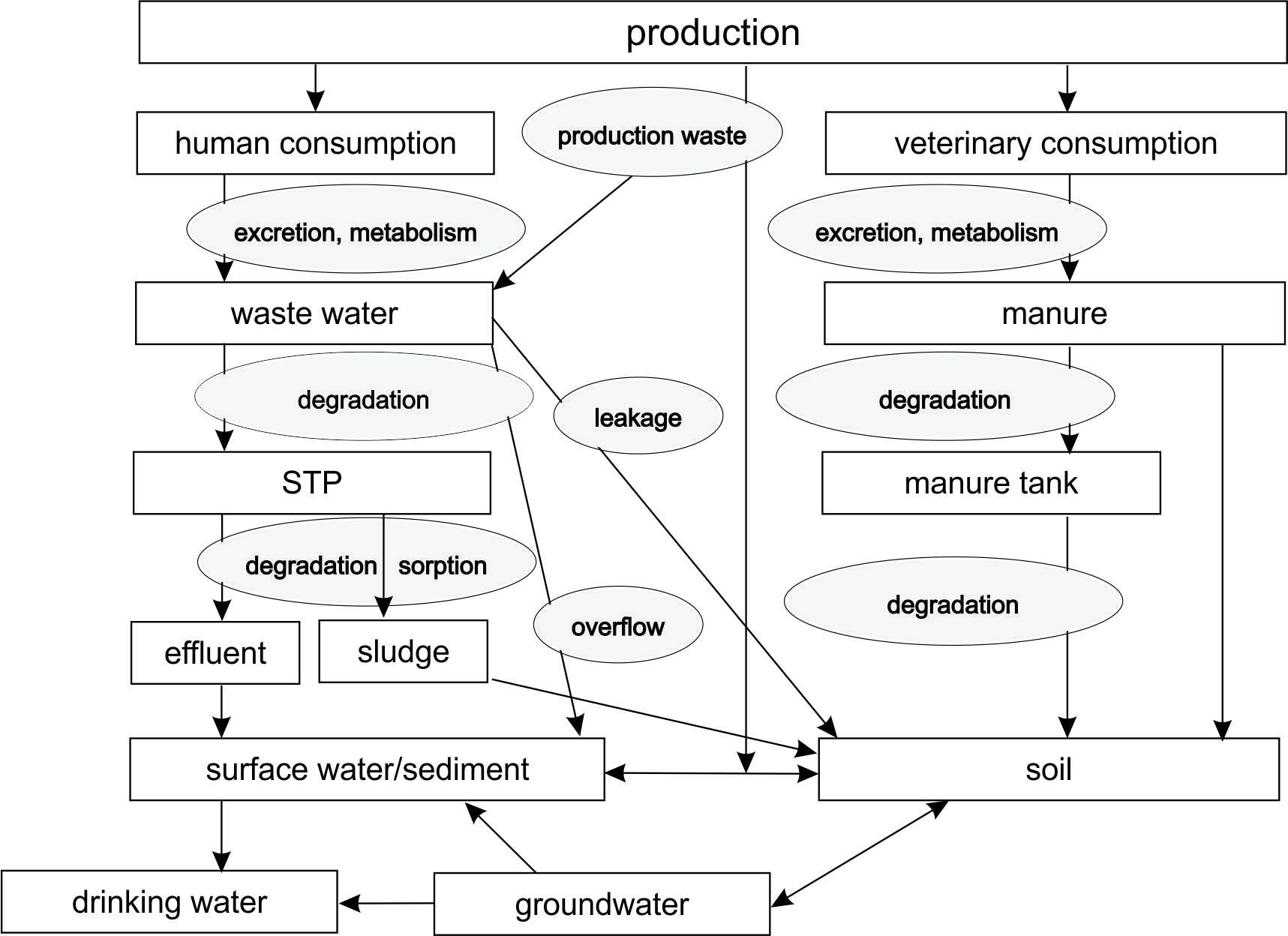
The active ingredients used in human and veterinary medicine partially overlap, however, the major fraction of pharmaceutically active substances in use are restricted to human consumption. In veterinary practice most of the applied pharmaceuticals are antibiotics and anti-parasitic agents, while in human medicine, pharmaceuticals to treat e.g. diabetes, pain, cardiovascular diseases, autoimmune disorders and neurological disorders make up a much larger portion of the pharmaceuticals in use. Worldwide pharmaceutical consumption has increased over the last century (several staggering numbers are summarized here). It is expected that the consumption will further increase due to a wider access to pharmaceuticals in developing countries. Additionally, demographic trends such as aging populations that is often seen in developed countries can also lead to increased consumption of pharmaceuticals, since older generations generally consume more pharmaceuticals than younger generations (van der Aa et al., 2011). The widespread and increasing use and their biological activity makes them relevant for environmental research. Below an overview is given on the emission, occurrence and fate(modeling) of pharmaceuticals in the environment.
Pharmaceuticals in the environment
Pharmaceuticals can enter the environment through various routes. Figure 1 gives and overview of emission routes of pharmaceuticals to the environment. Pharmaceutical are produced, transported to users (humans and/or animals), partially excreted by the users via urine and feces. For humans, the excrements are transport to wastewater treatment plants, septic tanks or directly emitted to soil or surface water. For animals, and especially livestock, manure contains residues of the pharmaceuticals. These pharmaceuticals end up in the environment when animals are grazing outside or when centrally collected manure is applied as fertilizer on arable land. Just like the pharmaceutical consumption, metabolism in the user (a human or an animal), the treatment and further application of communal wastewater and manure varies. Subsequently, emissions also vary between pharmaceuticals, countries and locations.
Concentrations of pharmaceuticals in the environment strongly vary, in Figure 2 concentration ranges of pharmaceuticals and some of their transformation products in the Meuse river and some tributaries are shown.
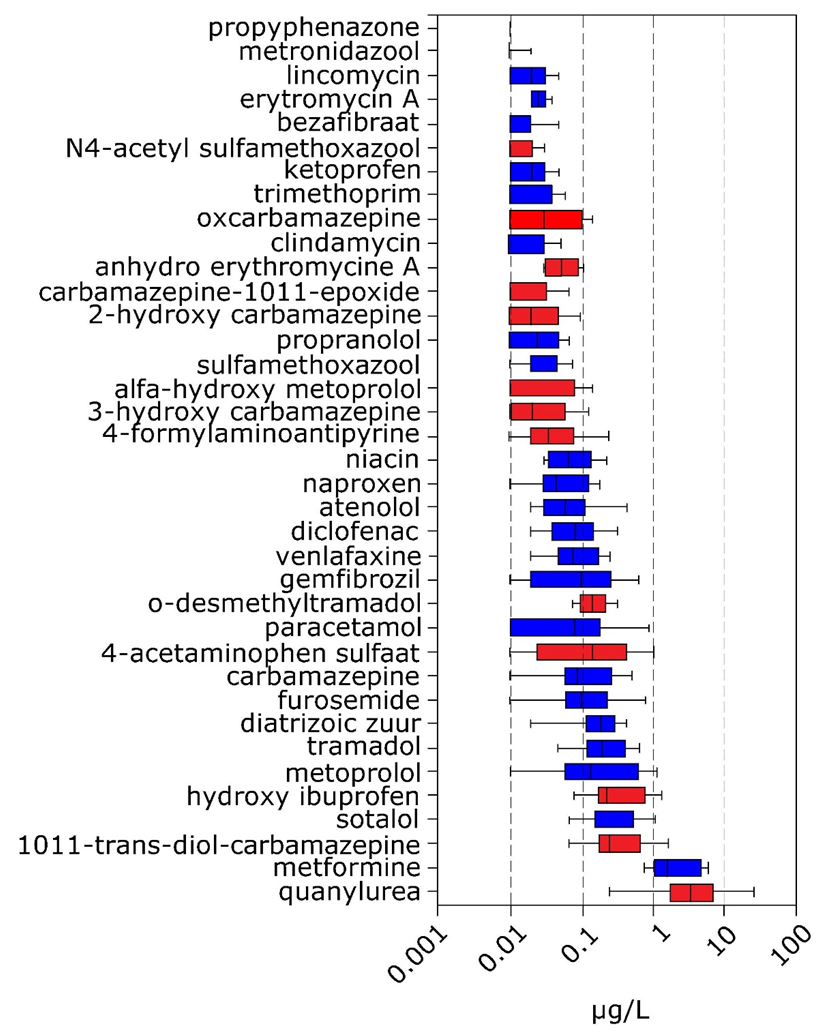
Properties of pharmaceuticals and their behavior and fate in the environment
Pharmaceuticals in use are developed for a wide array of diseases and therapeutic treatments. The chemical structures of these substances are therefore also very diverse, considering their size, structural presence of specific atoms, and physicochemical properties such as their hydrophobicity, aqueous solubility and ionization under environmentally relevant pH values, as shown for some examples in Figure 3.
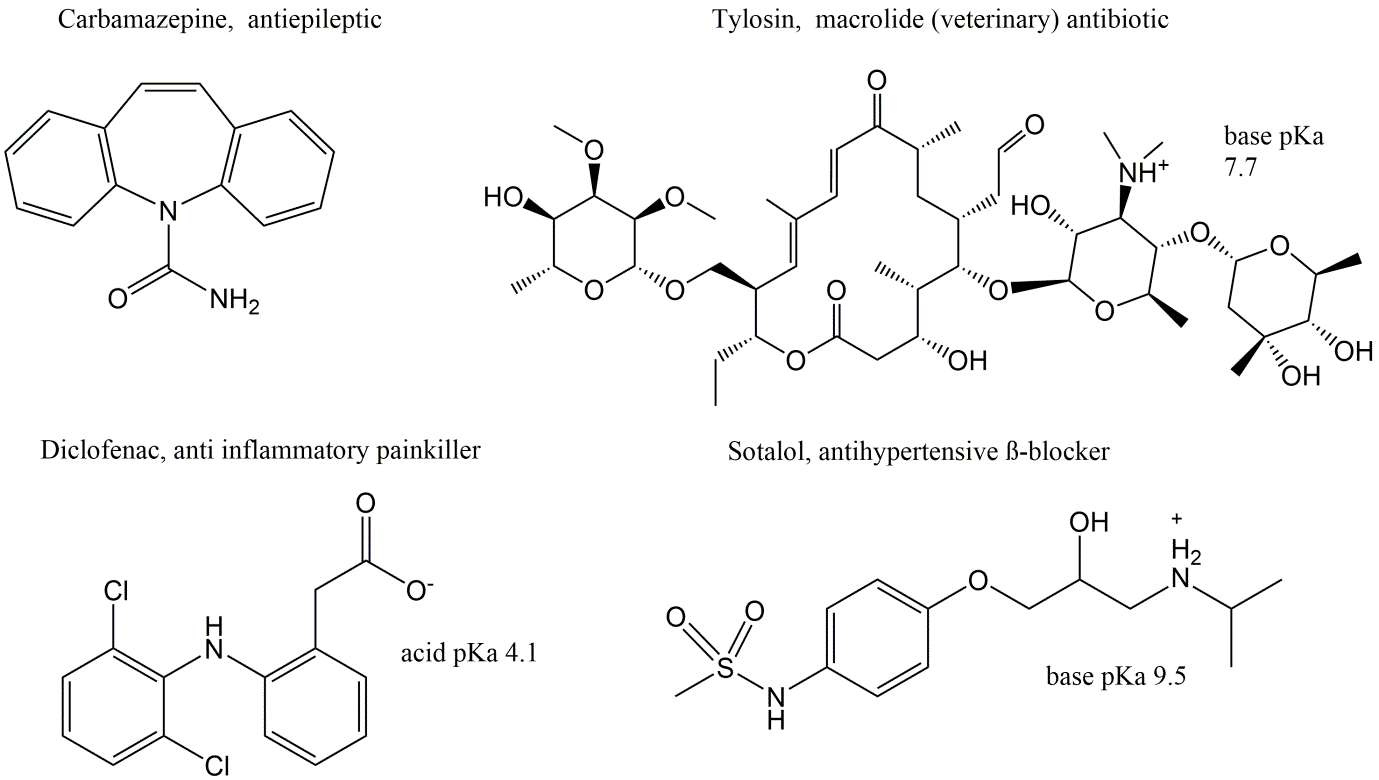
As a consequence of their structural diversity their environmental distribution and fate is also very variable. This makes it difficult to generalize the environmental behavior and fate of pharmaceuticals. Nevertheless, pharmaceuticals have generally certain properties in common:
These three generic properties also make them of environmental relevance since:
Occurrence and modelling of human and veterinary pharmaceuticals in the environment
Pharmaceuticals have been studied in the environment since the 1990s. Most studies have been performed in surface waters, but wastewater (effluents), groundwater, drinking water, manure, soil and sediments were also studied. Pharmaceuticals have been observed in all these matrices in concentrations generally varying from µg/L to sub ng/L levels (Aus der Beek et al., 2016, Monteiro and Boxall, 2010). Various studies have related environmental loads and related concentrations to human consumption data. Basically such -so called- mass balance or immision-emission balancing studies work according the following principle:
Modelling pharmaceuticals in the environment
Since the application of human pharmaceuticals, the consumption, is relatively well documented. Environmental concentrations can be related to consumption. This prediction works the best for the most persistent pharmaceuticals, since variations in loss factors are marginal for these pharmaceuticals. When loss factors become larger, they generally also become more variable, trough seasonal variations in use as well as variation in loss during wastewater treatment and loss processes in the receiving rivers, respectively. This makes the loads and concentrations of more degradable pharmaceuticals are more difficult to predict (Ter Laak et al., 2010).
Loads in a particular riverine system (such as a tributary of the river Meuse in the example below) can be predicted with a very simplified model. Here the pharmaceutical consumption over a selected period is multiplied by the factor of the selected pharmaceuticals that are excreted unchanged by the human body (ranging from 0 to 1)and the fraction that is able to pass the wastewater treatment plant (WWTP) (ranging from 0 to 1):
When this is related to actual measured concentrations and loads calculated from these numbers, the correlation between predicted and measured loads can be plotted. Various studies have shown that environmental loads can be predicted within a factor of 3 for most commonly observed pharmaceuticals (Ter Laak et al., 2014; Ter Laak et al., 2010; Alder et al., 2010; Oosterhuis et al., 2013).
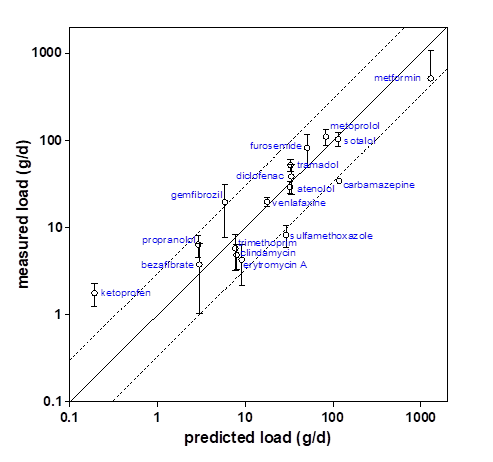
For veterinary pharmaceuticals this so called 'immision-emission balancing' is more difficult due to a number of reasons:
In a way the emissions and fate of veterinary pharmaceuticals is similar to emissions of pesticides used in agriculture, but then with a much poorer understanding on loads entering the system and the fate related to the various emission routes and emissions in combination with a complex matrix (urine, feces manure) (Guo et al., 2016). As a consequence, environmental fate studies of veterinary pharmaceuticals often describe specific cases, or cover laboratory studies to unravel specific aspects of the environmental fate of these pharmaceuticals (Kaczala and Blum, 2016; Kummerer, 2009).
Concluding remarks
Pharmaceuticals are commonly found in the environmental compartments such as surface water, soil, sediment and groundwater (Williams et al., 2016). Pharmaceuticals consist of a single or multiple active ingredients that have a specific biological activity. The therapeutic application and pharmacological mechanisms provide valuable information to evaluate the environmental hazard of these chemicals. Their physicochemical properties are of more relevance for the assessment of the environmental fate and exposure. The occurrence in the environment and the biological activity of this group of contaminants makes them relevant in environmental science.
References
Alder, A.C., Schaffner, C., Majewsky, M., Klasmeier, J., Fenner, K. (2010) Fate of [beta]-blocker human pharmaceuticals in surface water: Comparison of measured and simulated concentrations in the Glatt Valley Watershed, Switzerland. Water Research 44, 936-948
Aus der Beek, T., Weber, F., Bergmann, A., Hickmann, S., Ebert, I., Hein, A., Küster, A. (2016) Pharmaceuticals in the environment-Global occurrences and perspectives. Environ. Toxicol. Chem. 35, 823-835.
Guo, X.Y., Hao, L.J., Qiu, P.Z., Chen, R., Xu, J., Kong, X.J., Shan, Z.J., Wang, N. (2016) Pollution characteristics of 23 veterinary antibiotics in livestock manure and manure-amended soils in Jiangsu province, China. J. Environ. Sci. Health Part B Pestic. Food Contamin. Agric. Wastes 51 (6), 383-392
Kaczala, F., Blum, S.E. (2016) The occurrence of veterinary pharmaceuticals in the environment: A review. Curr. Anal. Chem., 12 (3), 169-182
Kümmerer, K. (2009) The presence of pharmaceuticals in the environment due to human use - present knowledge and future challenges. J. Environ. Manage., 90 (8), 2354-2366
Monteiro, S.C., Boxall, A.B.A. (2010) Occurrence and Fate of Human Pharmaceuticals in the Environment. Rev. Environ. Contam. Toxicol., 202, 53-154.
Oosterhuis, M., Sacher, F., ter Laak, T.L. (2013) Prediction of concentration levels of metformin and other high consumption pharmaceuticals in wastewater and regional surface water based on sales data. Sci. Total Environ. 442, 380-388
Ter Laak, T.L., Van der Aa, M., Houtman, C.J., Stoks, P.G., Van Wezel, A.P. (2010) Relating environmental concentrations of pharmaceuticals to consumption: A mass balance approach for the river Rhine. Environ. Intern. 36, 403-409
Ter Laak, T.L., Kooij, P.J.F., Tolkamp, H., Hofman, J. (2014) Different compositions of pharmaceuticals in Dutch and Belgian rivers explained by consumption patterns and treatment efficiency. Environ. Sci. Pollut. Res. 21, 12843-12855
Van der Aa, N.G.F.M., Kommer, G.J., van Montfoort, J.E. and Versteegh, J.F.M. (2011) Demographic projections of future pharmaceutical consumption in the Netherlands. Water Science and Technology, 825-832.
Williams, M., Backhaus, T., Bowe, C., Choi, K., Connors, K., Hickmann, S., Hunter, W., Kookana, R., Marfil-Vega, R., Verslycke, T. (2016) Pharmaceuticals in the environment: An introduction to the ET&C special issue. Environ. Toxicol. Chem. 35, 763-766.
Name three reasons why pharmaceuticals are relevant environmental contaminants?
Why is it easier to model human pharmaceuticals in the environment than veterinary pharmaceuticals?
Ibuprofen (anti-inflammatory) is discharged with the wastewater to the river. The following information is known about the use and fate of this drug.
- consumption per 1000 inhabitants of 1 g/d
- excretion by human 10 %
- removal by STP 68%
How much iboprufen is potentially discharged to the river in g/d ?
2.3.4. Drugs of abuse
Author: Pim de Voogt
Reviewer: John Parsons, Félix Hernández
Leaning objectives:
You should be able to:
- distinguish between licit and illicit drugs
- know what sources cause illicit drugs to show up in the environment
- understand what wastewater-based epidemiology is
Keywords: Cocaine, ecstasy, speed, cannabis, wastewater analysis
Introduction
Since about little more than a decade, drugs of abuse (DOA) and their degradation products have been recognized as emerging environmental contaminants. They are among the growing number of chemicals that can be observed in the aquatic environment.
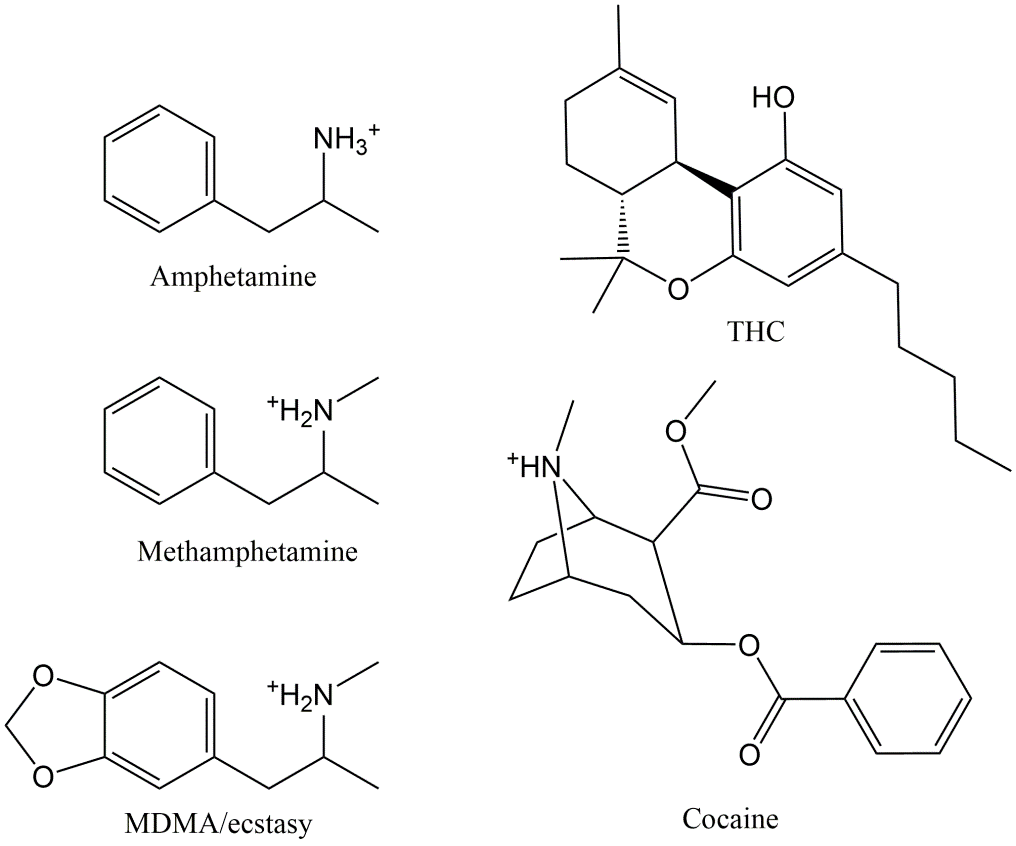
The residues of a major part of the chemicals used in households and daily life end up in our sewer systems. Among the many chemicals are cleaning agents and detergents, cosmetics, food additives and contaminants, pesticides, pharmaceuticals, and surely also illicit drugs. Once in the sewer, they are transported to wastewater treatment plants (WWTPs), where they may be removed by degradation or adsorption to sludge, or end up in the effluent of the plant when removal is incomplete.
The consumption of both pharmaceuticals and DOA has increased substantially over the last couple of decades as a result of several factors, including ageing of the population, medicalization of society and societal changes in life-style. As a result the loads in wastewater of drugs and their transformation products formed in the body after consumption have steadily increased. More recently, it has been observed that chemical waste from production sites of illicit drugs is being occasionally discharged into sewer systems, thereby dramatically increasing the loads of illicit drug synthesis chemicals and end products transported to WWTPs. As WWTPs are not designed to remove drugs, a substantial fraction of the loads may end up in receiving waters and thus pose a threat to both human and ecosystem health.
Drugs of Abuse (DOA)
Europe's most commonly used illicit drugs are THC (cannabis), cocaine, MDMA (ecstasy) and amphetamines. The structure of these drugs is given in Figure 1. Other important DOA include the opioids such as heroine and fentanyl, GHB, Khat and LSD.
Drugs of abuse are controlled by legislation, in The Netherlands by the Opium Act. The Opium Act encompasses two lists of substances. List one chemicals are called hard drugs while List II chemicals are known as soft drugs. Some narcotics are also being used for medicinal purposes, e.g., ketamine, diazepines, and one of the isomers of amphetamine. New psychoactive substances (NPS), also known as designer drugs or legal highs (because they are not yet controlled as they are not listed on the Opium Act lists), are synthesized every year and become available on the market in high numbers (see Figure 2).
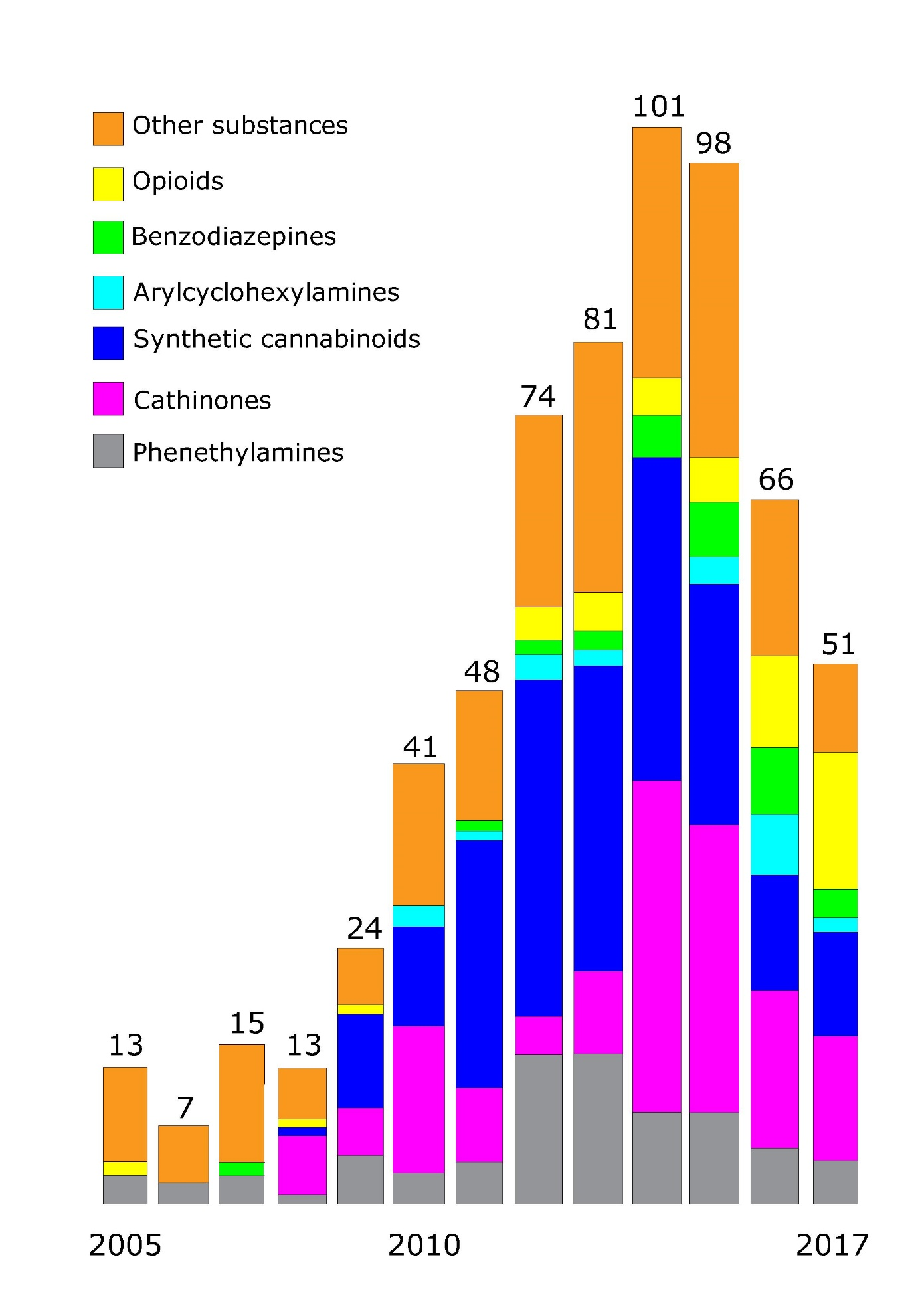
Wastewater-based epidemiology
Central sewage systems collect and pool wastewater from household cleaning and personal care activities as well as excretion products resulting from human consumption and thus contain chemical information on the type and amount of substances used by the population connected to the sewer. Drugs that are consumed are metabolized in the body and subsequently excreted. Excretion products can include the intact compounds as well as the transformation products, that can be used as biomarkers. An example of the latter is benzoylecgonine, which is the major transformation product of consumed cocaine. The collective wastewater from the sewer system carrying the load of chemicals is directed to the WWTP, and this wastewater influent can be sampled at the point where it enters the WWTP. By appropriate sampling of the influent during discrete time-intervals, e.g. 24 h, a so-called composite sample can be obtained and the concentrations of the chemicals can be determined. The volume of influent entering the WWTP is recorded continuously. Multiplying the observed 24 h average concentration of a compound with the total 24 h volume yields the daily load of the chemical entering the WWTP. This load can be normalised to the number of people living in the sewer catchment, resulting in a load per inhabitant. The loads of drugs in wastewater influents are usually expressed as mg.day-1.1000 inh-1. Normalised drug load data allow comparison between sewer catchments, such as shown in Figure 3. Obtaining chemical information about the population through wastewater analysis is known as Wastewater-based epidemiology, WBE (Watch the video). While WBE was developed originally to obtain data on consumption of DOA, the methodology has been shown to have a much wider potential: in calculating the consumption of e.g., alcohol, nicotine, NPS, pharmaceuticals and doping, as well as for assessing community health indicators, such as incidence of diseases or stress biomarkers.
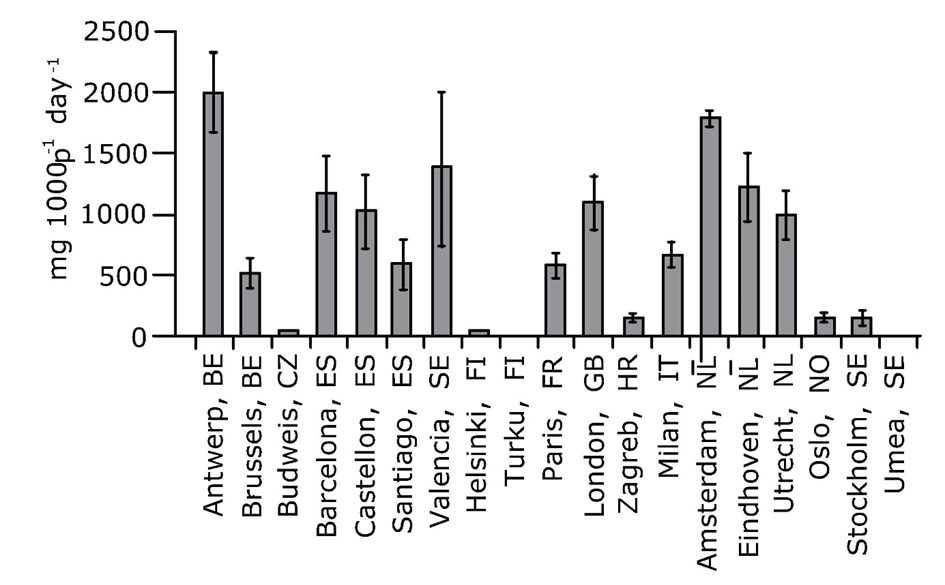
DOA and the environment
Barring direct discharges into surface waters or terrestrial environments, the major sources of DOA to the environment are WWTP effluents. Conventional treatment in municipal WWTPs has not been specifically designed to remove pharmaceuticals or DOA. Removal rates of DOA vary widely and depend on compound properties such as persistence and polarity as well as WWTP operational conditions and process configurations. Some DOA cross WWTPs almost unhindered, thus ending op in the receiving waters. Examples of the latter are MDMA and some diazepines (see Figure 4). Despite that several studies report the presence of DOA or their transformation products in surface waters, until now there is very little information about their aquatic ecotoxicity available in the scientific literature.
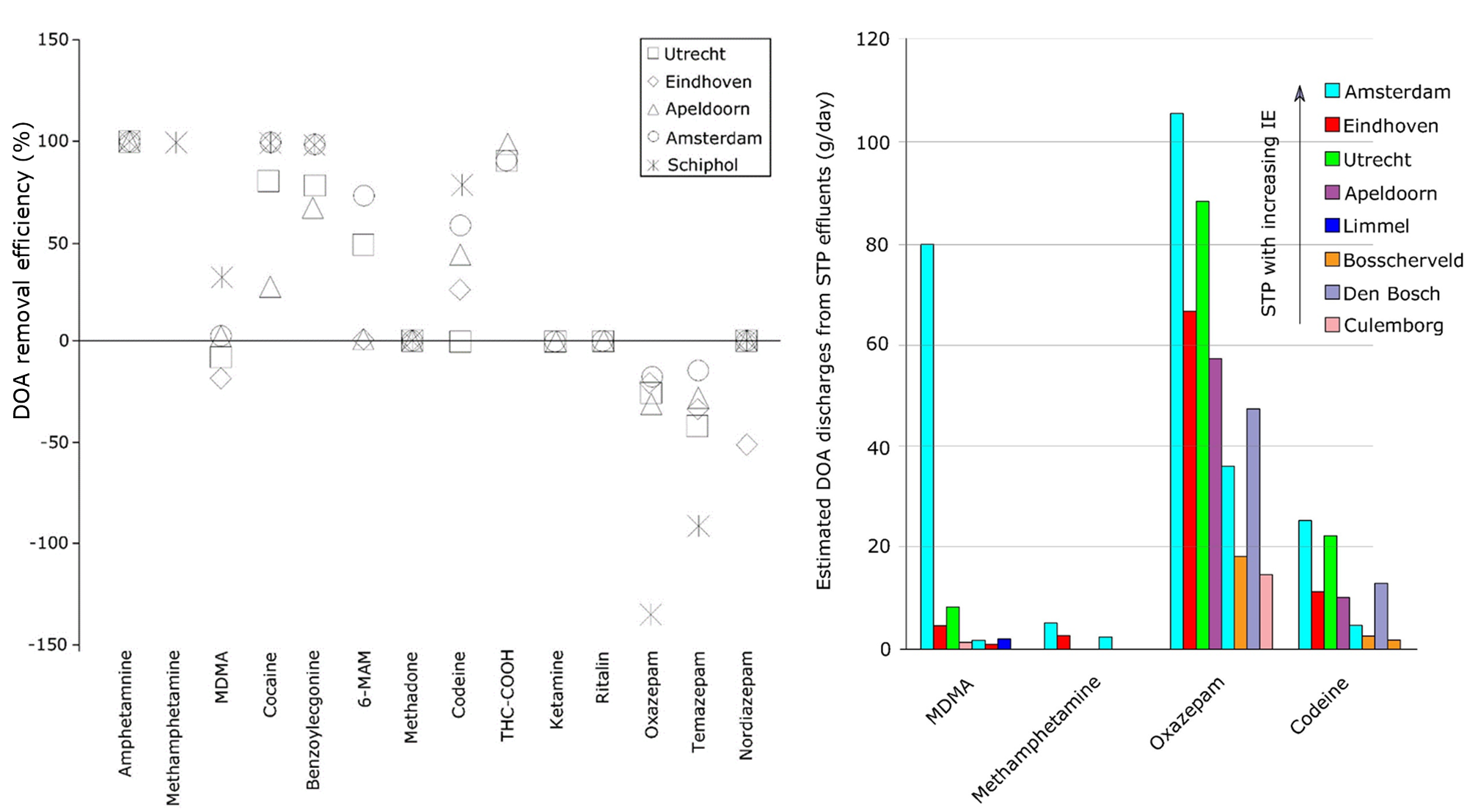
Recently, chemical waste from synthetic DOA manufacturing including their precursors and synthesis byproducts have been observed to be discharged directly into sewers. In addition, containers with chemical waste from DOA production sites have been dumped on soil or surface waters. Apart from solvents and acids or bases this waste often contains remainders of the synthesis products, which can then be dissipated in the aquatic environment or seep through the soil into groundwater.
Considering that DOA are highly active in the human body, it can be expected that some of them, in particular the more persistent ones, may exert some effects on aquatic biota when their levels increase in the aquatic environment.
References
Van der Aa, M., Bijlsma, L., Emke, E., et al. (2013). Risk assessment for drugs of abuse in the Dutch watercycle. Water Research 47(5), 1848-1857.
Bijlsma, L., Emke, E., Hernández, F., de Voogt, P. (2012). Investigation of drugs of abuse and relevant metabolites in Dutch sewage water by liquid chromatography coupled to high resolution mass spectrometry. Chemosphere 89(11), 1399-1406.
EMCDDA, European Drug Report 2018, Lisbon (www.emcdda.europa.eu/publications/edr/trends-developments/2018_en)
Thomas, K. V., Bijlsma, L., Castiglioni, S., et al. (2012). Comparing illicit drug use in 19 European cities through sewage analysis. Science of the Total Environment 432, 432-439.
One way of mapping the consumption volumes of psychoactive substances in a city is by chemical analysis of drug residues and biomarkers in wastewater. To assess the consumption volume of cocaine by a population the concentrations in wastewater of the substance benzoylecgonine (BE) are determined. Why is BE used instead of cocaine itself?
What possible sources can lead to the occurrence of an illicit drug in wastewater?
Can illicit drugs in wastewater pose a threat to the environment and if so, why?
2.3.5. Hydrocarbons
Author: Pim N.H. Wassenaar
Reviewer: Emiel Rorije, Eric M.J. Verbruggen, Jonathan Martin
Learning objectives:
You should be able to
- explain the diversity/variation in hydrocarbon structures.
- explain the specific and non-specific toxicological effects of several hydrocarbons.
Keywords: Hydrocarbons, Paraffins, Naphthenics, Aromatics
Introduction:
Hydrocarbons are a class of chemicals that only consist of carbon and hydrogen atoms. But despite their simplicity in building blocks, this group of chemicals consists of a wide variety of structures, as there are differences in chain length, branching, bonding types and ring structures. The main sources of hydrocarbons are crude oil and coal, which are formed over millions of years by natural decomposition of the remains of plants, animals or wood, and are used to derive products we are using on a daily basis, including fuels and plastics Other natural sources include natural burning (forest fires) and volcanic sources
Hydrocarbon classification
The major classes of hydrocarbons are paraffins (i.e. alkanes), naphthenics (i.e. cycloalkanes) and aromatics (Figure 1), and within these classes, several subclasses can be identified. Paraffins are hydrocarbons that do not contain any ring structures. Paraffins can be subdivided in normal (n-) paraffins, which do not contain any branching (straight chain), and iso-paraffins (i-), which do contain a branched carbon-chain. When alkanes include at least one carbon-carbon double bond, they are considered olefins (or alkenes).
Naphthenic and aromatic hydrocarbons both contain ring-structures but differ in the presence of aromatic or non-aromatic rings. The naphthenics and aromatics can be further specified based on their ring count; often mono-, di- and poly-ring structures are distinguished from each other. Of all these classes, the polycyclic aromatic hydrocarbons (PAHs) are the best-studied category in terms of all kinds of environmental aspects.
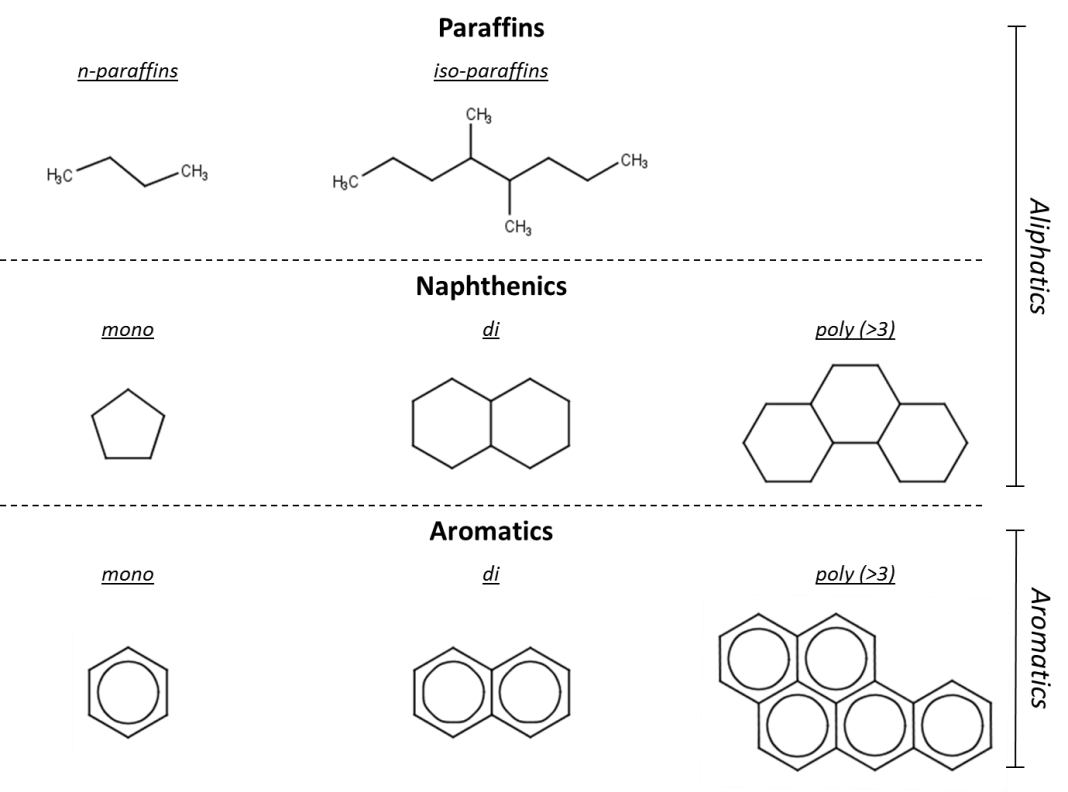
Besides the classes considered in Figure 1., combinations of these classes also exist. Naphthenic or aromatic structures with an alkane side chain are mostly still considered as naphthenic or aromatic hydrocarbons, respectively. However, when a non-aromatic-ring is fused with an aromatic-ring, the hydrocarbon is classified as a naphthenic-aromatic structure. Depending on the ring-count several subclasses can be identified, including naphthenic-mono-aromatics and naphthenic-poly-aromatics.
Concerns for human health and the environment
Because of their lack of polar functional groups, hydrocarbons are generally hydrophobic and, as a consequence, many are able to cause acute toxic effects in aquatic animals by a non-specific mode of action known as narcosis (or baseline toxicity). Narcosis is a reversible state of inhibited activity of membrane structures within the cells of organisms. Narcosis type toxicity is considered the minimum toxicity that any substance will be able to have, just by reaching concentration levels in the phospholipid bilayer of the cell membranes that disturb membrane transportation process. Hence the name "baseline" or minimum toxicity. When these events take place above a certain threshold, systemic toxicity can be observed in the organism, such as lethality. This threshold concentration is also known as the critical body residue (CBR) (Bradbury et al., 1989; Parkerton et al., 2000; Veith & Broderius, 1990).
Nevertheless, hydrocarbons can also have a more specific mechanisms of action, resulting in greater toxicity than baseline toxicity. For example, the toxicity of several PAHs increases in combination with ultraviolet radiation due to photo-induced toxicity. Photo-induced toxicity may be caused by photoactivation, in which a PAH is degraded into an oxidized product with a higher toxicity, or rather by photosensitization, in which reactive oxygen species (ROS) are formed due to an excited state of the PAHs (Figure 2) (Roberts et al., 2017). PAHs are especially vulnerable to photodegradation as their absorption spectrum falls within the range of wavelengths reaching the earth's surface (> 290 nm), which is not the case for most monoaromatic and aliphatic hydrocarbons (EMBSI, 2015). The photo-induced effects are of particular concern for aquatic species with transparent bodies, like zooplankton and early life stages, as more UV-light can penetrate into their organs and tissues (Roberts et al., 2017).
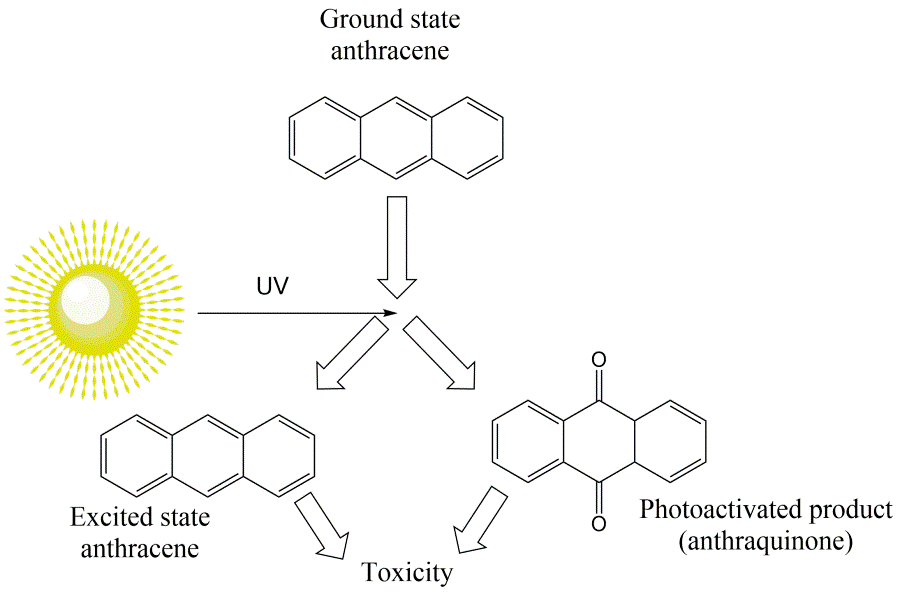
Several hydrocarbons are also able to cause genotoxicity and cancer upon exposure, including benzene, 1,3-butadiene and some PAHs. The carcinogenicity of PAHs is caused by biotransformation into reactive metabolites, specifically into epoxides which are the first step in oxidation of aromatic ring structures into dihydrodiol ring systems (Figure 3). In general, the biotransformation step increases the water solubility of the hydrocarbons (Phase I metabolism) and promotes subsequent conjugation and excretion (Phase II metabolism). However, several epoxide metabolites - more specifically the most stable aromatic epoxides - can reach the cell nucleus and covalently react with DNA, forming DNA adducts, and induce mutations (Figure 3). Ultimately, if not repaired such mutations can accumulate and may result in the formation of tumors (Ewa & Danuta, 2016). Specifically, PAHs with a bay-like region are of concern as biotransformation results in relatively stable reactive epoxides that are not accessible to epoxide hydrolase enzymes (Figure 3) (Jerina et al. 1980). Similar to PAHs, 1,3-butadiene and benzene are also able to cause cancer via the effects of their respective reactive metabolites (Kirman et al., 2010; US-EPA 1998).
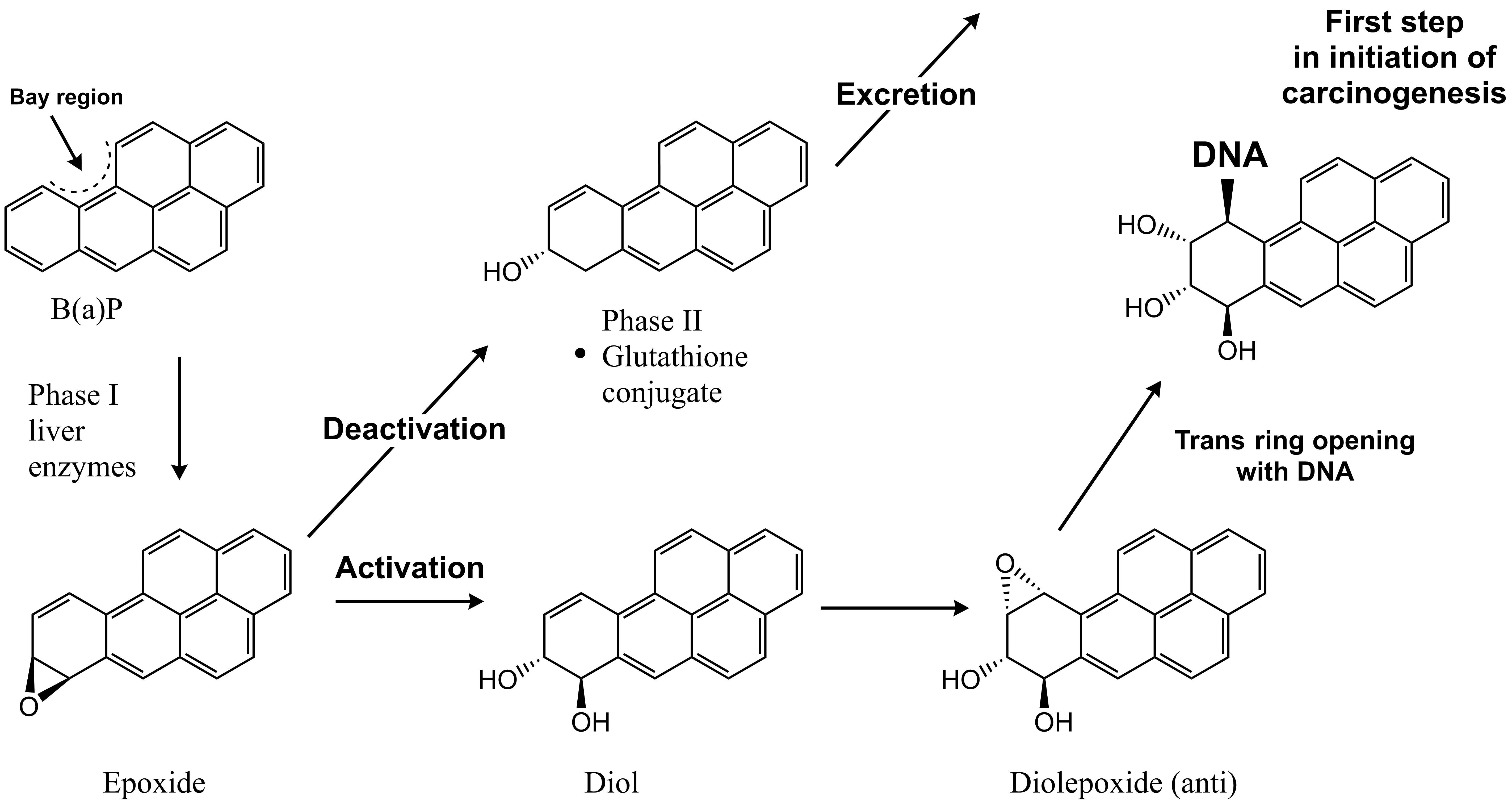
Besides their toxicity, some hydrocarbons such as the high molecular weight PAHs can be persistent in the environment and may accumulate in biota as a result of their hydrophobicity. It is therefore expected that internal concentrations are higher for such hydrocarbons and it is interesting that there is thus a relationship between narcosis and bioaccumulation potential. Consequently, these hydrocarbons might be of even greater concern.
Characterization of mixtures of hydrocarbons
As most research focused on specific hydrocarbons, including several PAHs, it is important to note that the biodegradation, bioaccumulation and toxicity potential of many hydrocarbons is still not fully known, such as for alkylated PAHs and naphthenics. As there is such a wide variety in hydrocarbon structures, it is impossible to assess the (potential) hazards of all hydrocarbons separately. Therefore, grouping approaches have been developed to speed up the risk assessment. Within a grouping approach, hydrocarbons can be clustered based on structural similarities. The underlying assumption is that all chemicals in a group are expected to have fairly similar physicochemical properties, and subsequently also fairly similar environmental fate and effect properties. As a result, such a group could potentially be assessed as if it is one single hydrocarbon.
The applicability of a hydrocarbon specific grouping approach, known as the Hydrocarbon Block Method (King et al., 1996), to assess the biodegradation and bioaccumulation potential of hydrocarbons is currently being investigated. Within this approach, all hydrocarbons are grouped based on their functional class (e.g. paraffin, naphthenic, aromatic) and the number of carbon atoms. The number of carbon atoms is thought to highly correlate with the boiling point of the hydrocarbons. An example matrix of the Hydrocarbon Block Method is presented in Figure 4. The composition of an oil substance could be expressed in such a matrix following GC-GC/MS analysis. Subsequently, the PBT-properties of the individual blocks could potentially be assessed by analyzing and extrapolating the PBT-properties of representative hydrocarbons for varying hydrocarbon blocks (see Figure 4).
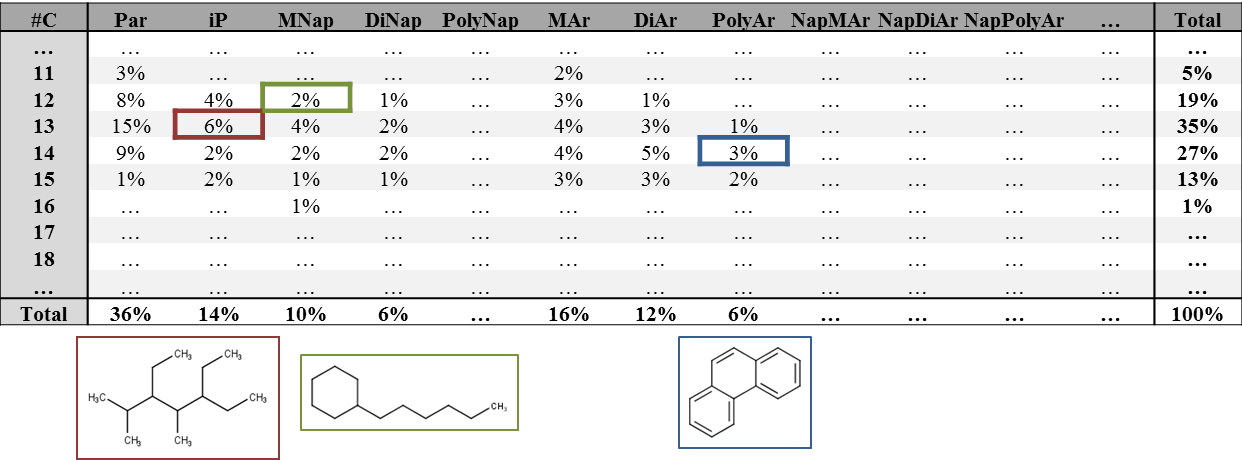
References
Bradbury, S.P., Carlson, R.W., Henry, T R. (1989). Polar narcosis in aquatic organisms. In Aquatic Toxicology and Hazard Assessment: 12th Volume. ASTM International.
EMBSI (2015). Assessment of Photochemical Processes in Environmental Risk Assessment of PAHs
Ewa, B., Danuta, M.Š. (2017). Polycyclic aromatic hydrocarbons and PAH-related DNA adducts. Journal of applied genetics 58, 321-330.
Homburger, F., Hayes, J.A., Pelikanm E.W. (1983). A Guide to General Toxicology. Karger/Base, New York, NY.
Jerina, D.M., Sayer, J.M., Thakker, D.R., Yagi, H., Levin, W., Wood, A.W., Conney, A.H. (1980). Carcinogenicity of polycyclic aromatic hydrocarbons: the bay-region theory. In Carcinogenesis: Fundamental Mechanisms and Environmental Effects (pp. 1-12). Springer, Dordrecht.
King, D.J., Lyne, R.L., Girling, A., Peterson, D.R., Stephenson, R., Short, D. (1996). Environmental risk assessment of petroleum substances: the hydrocarbon block method. CONCAWE report no. 96/52.
Kirman, C.R., Albertini, R.A., & Gargas, M.L. (2010). 1, 3-Butadiene: III. Assessing carcinogenic modes of action. Critical reviews in toxicology 40(sup1), 74-92.
Parkerton, T.F., Stone, M.A., Letinski, D. J. (2000). Assessing the aquatic toxicity of complex hydrocarbon mixtures using solid phase microextraction. Toxicology letters 112, 273-282.
Roberts, A.P., Alloy, M.M., Oris, J.T. (2017). Review of the photo-induced toxicity of environmental contaminants. Comparative Biochemistry and Physiology Part C: Toxicology & Pharmacology 191, 160-167.
US-EPA (1998). Carcinogenic Effects of Benzene: An Update. EPA/600/P-97/001F.
Veith, G.D., Broderius, S.J. (1990). Rules for distinguishing toxicants that cause type I and type II narcosis syndromes. Environmental Health Perspectives 87, 207.
Mention at least three aspects that influence the variation in hydrocarbon structures?
To which hydrocarbon class (or Hydrocarbon Block) do the following structures belong?
1.
2.
3.
What kind of toxic effects can be observed for polycyclic aromatic hydrocarbons?
2.3.6. CFCs
(draft)
Authors: Steven Droge
Reviewer: John Parsons
Leaning objectives:
You should be able to:
- realize what the ozone layer depletion was all about
- understand why certain replacement chemicals are still problematic
Keywords: Ozone layer, refrigerator, volatile chemicals, spray cans, radicals
Introduction
CFCs (chlorofluorocarbons) were very common air pollutants in the 20th century because they were the basic components of refrigerants and air conditioning, propellants (in spray can applications), and solvents, since the 1930s. They are still very common air pollutants, because they are very persistent chemicals, and emissions do still continue. In the first years as refrigerants, they replaced the much more toxic components ammonia (NH3), chloromethane (CH3Cl), and sulfur dioxide (SO2). Particularly the CFCs leaking from old refrigerating systems in landfills and waste disposal sites caused high emissions into the environment. Typically, these volatile CFC chemicals are based on the smallest carbon molecules methane (CH4), ethane (C2H6), or propane (C3H8). All hydrogen atoms in these CFC molecules are replaced by a mixture of chlorine and fluorine atoms.
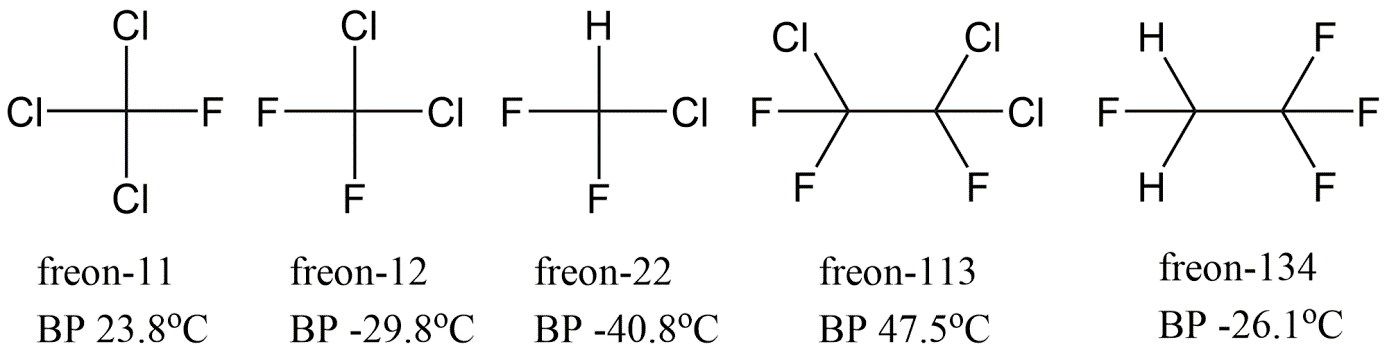
CFCs are less volatile than their hydrocarbon analogue, because the halogen atoms polarize the molecules, which causes stronger intermolecular attractions. Depending on the substitution with Cl or F, the boiling point can be tuned to the desired point for refrigerating cooling processes. The CFCs are also much less flammable than hydrocarbon analogues, making them much safer in all kinds of applications.
Naming of CFCs
CFCs were often known by the popular brand name Freon. Freon-12 (or R-12) for example stands for dichlorodifluoromethane (CCl2F2, boiling point -29.8 °C, while methane has -161 °C), as shown in Figure 1. The naming reflects the amount of fluor atoms as the most right number. The next value to the left is the number of hydrogen atoms plus 1, and the next value to the left is the number of carbon atoms less one (zeroes are not stated), and the remaining atoms are chlorine. Accordingly, Freon-113 could apply to 1,1,2-trichloro-1,2,2-trifluoroethane (C2Cl3F3, boiling point 47.7 °C, while ethane has -161 °C). The structure of any Freon-X number can also be derived from adding +90 to the value of X, so Freon-113 would give a value of 203. The first numerical is the number of C (2), the second numerical H (0), the third numerical F (3), and the remaining substitutions are by chlorine (C2X6 gives 3 chlorines).
The reason CFC depletes the ozone layer
The key issue with CFC emissions is the reaction under influence of light ("photodegradation") that ultimately reduces ozone concentrations ("ozone depletion") in the upper atmosphere ("stratosphere"). Ozone absorbs the high energy radiation of the solar UV-B spectrum (280-315nm), and the ozone layer therefore prevents this to reach the Earth's surface. The even more energetic solar UV-C spectrum (100-280nm) is actually causing the formation of ozone (O3) when reacting with oxygen (O2), as shown in Figure 2. Under the influence of intense light-energy in the upper atmosphere, CFC molecules can disintegrate into two highly reactive radicals (molecules with a free electron . ), for Freon-11:
It is the radical Cl. that catalyzes the conversion of ozone back into O2. The environmentally relevant role of the fluorine atoms in CFCs is that they make these chemicals very persistent after emission, because the C-F bond is one of the strongest covalent bonds known. With half-lives up to >100 years, high CFC levels can reach the upper atmosphere. James Lovelock was the first to detect the widespread presence of CFCs in air in the 1960s, while the damage caused by CFCs was discovered only in 1974. Another undesirable effect of CFC in the stratosphere is that they are a much more potent greenhouse gases than CO2.
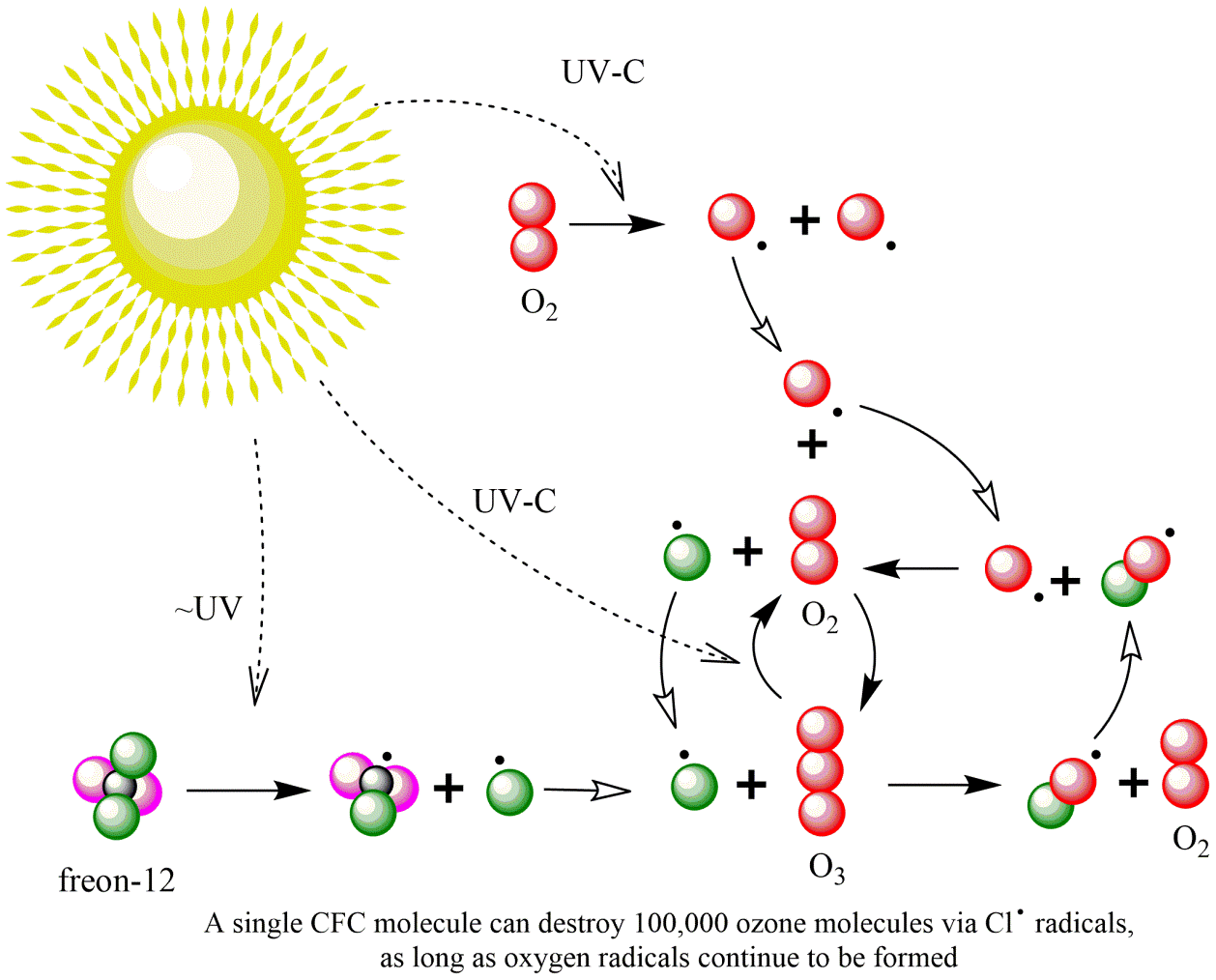
CFC replacements.
In 1978 the United States banned the use of CFCs such as Freon in aerosol cans. After several years of observations of the ozone layer depletions globally (Figure 3), particularly above Antarctica, the Montreal Protocol was signed in 1987 to drastically reduce CFC emissions worldwide. CFCs were banned by the late 1990s in most EU countries, and e.g. in South Korea by 2010. Due to the persistency of CFCs it may take until 2050-2070 before the ozone layer will return to 1980 levels (which were bad already).
The key damaging feature of CFCs in terms of ozone depletion is their persistency, so that emissions reach and build up in the stratosphere (starting from 20km above the equator, but only at 7km above the poles). CFC replacement molecules were initially found simply by adding more hydrogens in the CFC structures and somewhat less Cl (HCFCs), but fractions still contributed to Cl. radicals. Later alternatives lack the chlorine atoms and have even shorter lifetimes in the lower atmosphere, and simply cannot form the Cl radicals. These "hydrofluorocarbons" (HFCs) are currently common in automobile air conditioners, such as Freon-134 (do the math to see that there is no Cl, boiling point -26.1 °C).
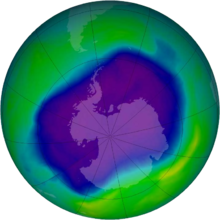
Still, HCFC as well as HFCs are still very potent greenhouse gasses, so the worldwide use of such chemicals remains problematic and gives rise to new legislations, regulations, and searches for alternatives. R-410A (which contains only fluorine) is becoming more widely used but is 1700 times more potent than CO2 as greenhouse gas, equal to Freon-22. Simple hydrocarbon mixtures such as propane/isobutane are already used extensively in mobile air conditioning systems, as they have the right thermodynamic properties for some uses and are relatively safe. Unfortunately, we did not have the technological skills, nor the awareness to apply this back in the 1930s.
2.3.7. Cosmetics/personal care products
(draft)
Author: Mélanie Douziech
Reviewers: John Parsons
Learning objectives:
You should be able to:
- Define what personal care products and cosmetics are
- Explain how chemicals from personal care products end up in the environment
- Cite and describe some of the most common chemicals found in personal care products
Keywords: wastewater, chemical function, surfactants, microbeads
Introduction
Personal care products (PCPs) cover a large range of products fulfilling hygiene, health, or beauty purposes (e.g. shampoo, toothpaste, nail polish). They are categorized into oral care, skin care, sun care, hair care, decorative cosmetics, body care and perfumes. Overall, most PCPs are classified as cosmetics and regulated accordingly. In the European Union (EU) the Cosmetic Regulation governs the production, safety of ingredients and the labelling and marketing of cosmetic products. The United States of America (USA), on the other hand, have a narrower definition of cosmetics so that products not fulfilling the definition are regulated as pharmaceuticals (e.g. sunscreen) (Food and Drug Administration, 2016).
PCPs come in a range of formats (e.g. liquids, bars, aerosols, powders) and typically contain a wide range of chemicals, each fulfilling a specific function within the product. For example, a shampoo can include cleansing agents (surfactants), chemicals to ensure product stability (e.g. preservatives, pH adjusters, viscosity controlling agents), diluent (e.g. water), perfuming chemicals (fragrances), and chemicals to influence the product's appearance (e.g. colourants, pearlescers, opacifiers). The chemicals present in PCPs ultimately enter the environment either through air during direct use, such as the propellants in aerosols, or through wastewater via down the drain disposal following product use (e.g. shower products, toothpaste). The release of PCP chemicals into the environment needs to be monitored and the safety of these chemicals understood in order to avoid potential problems. In developed countries, the use of wastewater treatment plants (WWTPs) is key to effectively removing the PCP chemicals and other pollutants from wastewater prior to their release to rivers and other watercourses. The removal mechanisms occurring in WWTPs include biodegradation, sorption onto solids, and volatilization to the air. The extent of removal is influenced by the physicochemical properties of the chemicals and the operational conditions of the WWTPs. In regions where wastewater treatment is lacking, the chemicals in PCPs enter the environment directly.
The wide scale daily use of PCPs and the associated large volumes of chemicals released explain why they are scrutinized by environmental protection agencies and regulatory bodies. The following sections will briefly review some of the classes of chemicals used in PCPs by describing their behavior in the environment and their potential effect on ecosystems.
Cleansing agents - surfactants
Surfactants are an important and widely used class of chemicals. They are the key components of many household cleaning agents as well as PCPs, such as shampoos, soaps, bodywash and toothpaste, because of their ability to remove dirt. These dirt-removing properties also make surfactants inherently toxic to aquatic organisms. The biodegradability of surfactants is a key legal requirement for their use in PCPs to minimize the likelihood of unsafe levels in the environment. Different types of surfactants exist and are often classified based on their surface charge. Anionic surfactants, which carry a negative surface charge, interact and help remove positively charged dirt particles from surfaces such as hair and skin. Sodium lauryl sulfate is a typical example of an anionic surfactant used in PCPs. Cationic surfactants, such as cetrimonium chloride, are positively charged and may be used as hair conditioning agents to make hair shinier or more manageable. Non-ionic surfactants (uncharged), such as cetyl alcohol, help formulate products or increase foaming. Amphoteric surfactants, such as sodium lauriaminodipropionate, carry both positive and negative charges and are commonly used to counterbalance the potentially irritating properties of anionic surfactants.
Fragrances
Fragrances are mixtures of often more than 20 perfumery chemicals used to provide the smell of PCPs. Typically, fragrances are present at very low levels in most PCPs (below 0.01%) so that their exact compositions are not disclosed. Disclosed, however, are any allergens present in the fragrance to help dermatologists and consumers avoid certain fragrance chemicals. Despite the wish to protect trade secrets, a recent trend increasingly sees companies disclose the full fragrance compositions of their products on their websites (e.g. L'Oréal, Unilever). Well-known examples of fragrances include hexyl cinnamal, linalool, and limonene. Potential concerns about the ecotoxicological impact of fragrances have arisen on the one hand because of a lack of disclosure of fragrance formulations and on the other hand because of the detection of certain persistent fragrances in the environment (e.g. nitromusks).
Preservatives
Preservatives are usually added to PCPs containing water for their ability to protect the product from contamination by bacteria, yeasts, and molds during storage or repeated use. Given their targeted action against living organisms, the use of preservative in chemical products including PCPs is under constant scrutiny. For example, in 2016 and 2017, the European Commission tightened the regulation around the use of methylisothiazolinone in cosmetics products due to human safety concerns. Other preservatives that have been restricted in use, because of both human safety and environmental safety concerns (e.g. endocrine disruption effects), include certain types of parabens and triclosan.
UV filters
UV filters are used in sunscreen products as well as in other PCPs such as foundation, lipstick, or moisturizing cream to protect users from UV radiation. UV filters can be organic or inorganic. Inorganic UV filters, like titanium oxide and zinc oxide, form a physical boundary protecting the skin from UV radiation. Organic UV filters, on the other hand, protect the skin by undergoing a chemical reaction with the incoming UV radiation. Organic UV filters commonly found in PCPs include butyl methoxydibenzoylmethane, ethylhexyl methoxycinnamate, and octocrylene. Organic UV filters are poorly biodegradable and have the potential to accumulate in organisms. Further, a number of organic UV filters have been shown to be toxic to coral organisms in laboratory tests. They are suspected to cause coral bleaching by, for example, promoting viral infections but research is still on-going to understand their potential ecotoxicological effects at realistic environmental concentrations.
Volatile chemicals
Certain chemicals used in PCPs are highly volatile and may end up in the air following product use. Examples include propellants, such as propane butane mixes or compressed air/nitrogen, used in aerosols to apply ingredients in hairsprays or deodorants and antiperspirants. Fragrances also volatilize when the product is applied to skin or hair to provide smell. Volatile silicones, chemicals used to assist the deposition of ingredients in liquids and creams, are another example of chemicals emitted to air upon PCP use.
The special case of plastic microbeads
Plastic microbeads, with a diameter smaller than 5mm, have been used in PCPs such as face scrubs or shower gels for their scrubbing and cleansing properties. The growing concern about plastic pollution in water has drawn attention to the use of microbeads in PCPs. As a result, a number of initiatives were launched both to highlight the use of plastic microbeads and to encourage replacement with natural alternatives. An example thereof is the "Beat the microbead" coalition (https://www.beatthemicrobead.org/) sponsored by the United Nations Environment Program, launched to help consumers identify and avoid PCPs containing microbeads. Such initiatives together with voluntary commitments by industry have led to a large decrease in the use of microbeads in wash-off cosmetic products: In the EU, for example, the use of microbeads in wash-off products was reduced by 97% from 2012 to 2017. Legislation to restrict the use of microbeads has also recently been put in place. In the USA microbeads in PCPs were banned in July 2017 and a number of EU countries (e.g. United Kingdom, Italy) have also banned their use in wash-off products.
Further reading
For more information on PCP chemicals and their function in products, please see (European commission 2009; Grocery Manufacturers Association 2017).
For more information on the different types of surfactants, please see Tolls et al. (2009) and Section 2.3.8.
Manova et al. (2013) list the different types of UV filters.
The report of Scudo et al. (2017) gives more information on the use of microplastics in Europe.
References
European Commission (2019). Cosing. 2009 03.2019]; Available from: http://ec.europa.eu/growth/tools-databases/cosing/.
Food and Drug Administration (2016). Are All "Personal Care Products" Regulated as Cosmetics? [cited 2019 03]; Available from: https://www.fda.gov/forindustry/fdabasicsforindustry/ucm238796.htm.
Grocery Manufacturers Association (2017). Smartlabel. [cited 2017 11]; Available from: http://www.smartlabel.org/.
Manova, E., von Goetz, N., Hauri, U., Bogdal, C., Hungerbuhler, K. (2013). Organic UV filters in Personal Care Products in Switzerland: A Survey of Occurrence and Concentrations. International Journal of Hygiene and Environmental Health 216, 508-514.
Scudo, A., Liebmann, B., Corden, C., Tyrer, D., Kreissig, J., Warwick, O. (2017). Intentionally Added Microplastics in Products. in: Limited A.F.W.E.a.I.U., ed. United Kingdom
Tolls, J., Berger, H., Klenk, A., Meyberg, M., Müller, R., Rettinger, K., Steber, J. (2009). Environmental safety aspects of Personal Care Products - a European perspective. Environmental Toxicology and Chemistry 28, 2485-2489.
How do chemicals found in cosmetics end in the environment?
Why are surfactants a concern for environmental toxicity?
How did personal care products contribute to the microplastic pollution in the water?
2.3.8. Detergents and surfactants
Author: Steven Droge
Reviewer: Thomas P. Knepper
Leaning objectives:
You should be able to:
- explain why surfactants remove dirt
- discuss historical progress on surfactant biodegradability
- describe the different types of common surfactants.
- describe examples of how surfactants enter the environment.
Keywords: amphiphilic chemicals, micelle formation, biodegradability
Introduction
Surface active agents ("surf-act-ants") are a wide variety of chemicals produced in bulk volumes (>10.000 tonnes annually) as a key ingredient in cleaning products: detergents. Typical for surfactants is that they have a hydrophobic tail and a hydrophilic head group (Figure 1).
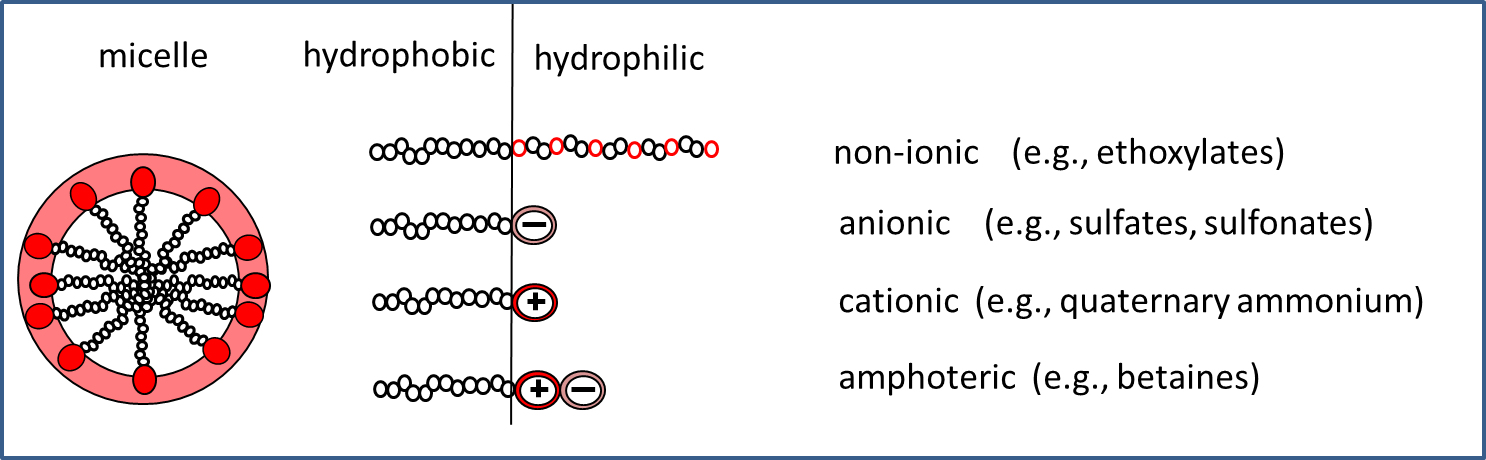
At relatively high concentrations in water (typically >10-100 mg/L), surfactants spontaneously form aggregated structures called micelles (Figure 1), often in spheres with the hydrophobic tails inward and the hydrophilic head groups towards the surrounding water molecules. These micelle super-structures allow surfactants to dissolve grease and dirt from e.g. textile or dishes into water, which can then be flushed away. Besides this common use of surfactants, their amphiphilic (i.e., both hydrophilic and lipophilic) properties allow for a versatile use in our modern world:
• During the large 2010 oil spill in the Mexican Gulf, enormous volumes (>6700 tonnes) of several types of surfactant formulations (e.g. "Corexit") were used to disperse the constant stream of oil leaking from the damaged deep water well into small dissolved droplets, in order to facilitate microbial degradation and prevent the formation of floating oil slabs that could ruin coastal habitats.
• The ability of a layer of surfactants to maintain hydrophobic particles in solution is a key process in many products, such as paints and lacquers.
• The ability to emulsify dirt particles is a key feature in process fluids during deep drilling in soil or sediment.
• Fabric softners, and hair conditioners, have cationic surfactants as key ingredients that stick with the positively charged head groups onto the negatively charged fibers of your towel or hair. After the final flushing, these cationic surfactants still stick on the fibers and because of the hydrophobic head groups sticking out make these materials feel soft and smooth. Often only during the next washing event (with anionic or nonionic surfactants) the cationic surfactants are flushed off the fibers.
• Many cationic surfactants have biocidal properties at relatively low concentrations and are therefore used in a few percent in many cosmetic products as preservatives, e.g. in cosmetics, or used to kill microbes in food processing, antibacterial hand wipes or during swimming pool cleaning. Examples are chloride salts of benzalkonium, benzethonium, cetylpyridinium.
• Surfactants lower the surface tension of water, and therefore are used (as "adjuvants") in pesticide products to facilitate the droplet formation during spraying and to improve contact of the droplets with the target leaves in case of herbicides. Examples are fluorinated surfactants, silicone based surfactants (Czajka et al. 2015), and polyethoxylated tallow amine (POEA) used for example in the glyphosate formulation Roundup.
The hydrophobic tail of surfactants is mostly composed of a chain of carbon atoms, although also fluorinated carbon (-CF2-) chains or siloxane (Si(CH3)3-O-[..]-Si(CH3)3) chains are also possible.
The first bulk volume produced surfactants for washing machines were branched anionic alkylbenzenesulfonates (ABS) and alkylphenolethoxylates (APEO), with the hydrocarbon source obtained from petroleum. Because of the variable petroleum source, these chemicals are often complex mixtures. However, hydrophobic branched alkylchains are poorly biodegraded, and the constant disposal of these surfactants into the waste water caused very high environmental concentrations, often leading to foaming rivers (Figure 2).
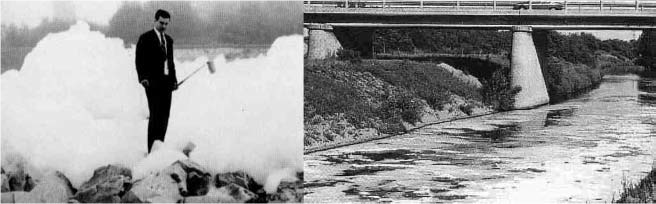
Surfactant producers 'voluntarily' switched to carbon sources such as palm oil or controlled polymerization of petrol-based ethylene, that could be used to generate surfactants with linear alkyl chains: linear alkylbenzenesulfonate (LAS) and alcohol ethoxylates (AEO). Some surfactants have the hydrophilic headgroup attached to two carbon chains, such as the anionic docusate (heavily used in the BP oil spill) and the cationic dialkyldimethylammonium chemicals. Common detergent surfactants are nowadays designed to pass ready biodegradability tests (>60% mineralisation to CO2 within a 10 d window following a lag phase, in a 28 d test). Early examples of fabric softners are double chain (dialkyl)dimethylammonium surfactants, but the environmental persistency of these compounds (DODMAC and DHTDMAC, see e.g. EU and RIVM-reports) has led to a large replacement by diesterquats (DEEDMAC), which degrade more rapidly through the weak ester linkages of the fatty acid chains (Giolando et al. 1995). A switch to sustainable production of the carbon sources is ongoing. Whereas petroleum based ethylene oil was mostly used, it is being replaced increasingly by the linear fatty acid carbon chains from either palm-oil (mostly C16/C18), coconu oil (mostly C12/C14), but also such raw materials needs to be as sustainably derived as possible.
The hydrophilic headgroups can vary extensively. Nonionic surfactants can have a simple polar functional group (amide), glucose based (polyglycoside), or contain a variable lengths of repetitive ethoxlyate and/or propoxylate units. Because the ethoxylation process is difficult to control, such surfactants are often complex mixtures. Anionic surfactants are often based on sulfate (SO4-) or sulfonate (SO4-), but also phosphonate and carboxylates are common. A key difference between anionic surfactants is that sulfate and sulfonates are fully anionic (pKa ~<0) over the entire environmental pH range (pH4-9), while carboxylates are weaker acids that are still partially neutral species (pKa ~5). Most cationic surfactants are based on permanently charged quaternary ammonium headgroups (R-(N+)(CH3)3), although several ionizable amine groups are applied in cationic surfactants too (e.g., diethanolamines).
The key ingredient property of most surfactants is the critical micelle concentration (CMC), which defines the dissolved concentration above which micellar aggregates start to form that can remove grease or fully emulsify particles. The CMC decreases proportionally with the hydrophobic tail length, and this means that with longer tails, you need less surfactant to start to form micelles. However, with increasing hydrophobic tails anionic surfactants more readily precipitate with dissolved inorganic cations such as calcium. Also, surfactant toxicity increases proportionally with hydrophobic tail lengths. If the alkyl chain is too long, the surfactant may bind strongly to all kinds of surfaces and not be available for micelle formation. The optimum hydrophobic chain length is thus often a balance between the desired properties of the surfactant and several critical processes that influence the efficiency and risk of surfactants.
References
Kümmerer, K. (2007). Sustainable from the very beginning: rational design of molecules by life cycle engineering as an important approach for green pharmacy and green chemistry. Green Chemistry 9, 899-907 . DOI: 10.1039/b618298b
Giolando, S.T., Rapaport, R.A. , Larson, R.J., Federle, T.W., Stalmans, M., Masscheleyn P. (1995). Environmental fate and effects of DEEDMAC: A new rapidly biodegradable cationic surfactant for use in fabric softeners. Chemosphere 30, 1067-1083. DOI: 10.1016/0045-6535(95)00005-S
Czajka, A., Hazell, G., Eastoe, J. (2015). Surfactants at the Design Limit. Langmuir 31, 8205−8217. DOI: 10.1021/acs.langmuir.5b00336
Scientific Committee on Toxicity, Toxicity and the Environment (CSTEE) (2001). Opinion on the results of the Risk Assessment of: Dimethyldioctadecylammonium chloride (DODMAC). EU-report C2/JCD/csteeop/DodmacHH22022002/D(02) https://ec.europa.eu/health/archive/ph_risk/committees/sct/documents/out143_en.pdf
Van Herwijnen, R. (2009). Environmental risk limits for DODMAC and DHTDMACRIVM Letter report 601782029 - https://www.rivm.nl/bibliotheek/rapporten/601782029.pdf
Try to look up toxicity literature for the pesticide formulation Roundup and compare the apparent toxicities of the active ingredient glyphosate and the adjuvant surfactant to nontarget organisms. What do you notice?
Surfactants do not just accumulate at the air-water interface, but also at water-solvent interfaces. The octanol-water partition coefficient is a critical property for risk assessment, but can you provide reasons why this property is problematic to be derived and applied for surfactants?
Although detergents are designed to be readily biodegradable, some compounds appear to be pseudo-persistent as they are still found at detectable levels near the outflow of sewage treatment effluent pipes. What do you think is meant by this?
2.3.9. Food and Feed Additives
In preparation