8.7: Standard Enthalpy of formation
- Page ID
- 369495
\( \newcommand{\vecs}[1]{\overset { \scriptstyle \rightharpoonup} {\mathbf{#1}} } \)
\( \newcommand{\vecd}[1]{\overset{-\!-\!\rightharpoonup}{\vphantom{a}\smash {#1}}} \)
\( \newcommand{\id}{\mathrm{id}}\) \( \newcommand{\Span}{\mathrm{span}}\)
( \newcommand{\kernel}{\mathrm{null}\,}\) \( \newcommand{\range}{\mathrm{range}\,}\)
\( \newcommand{\RealPart}{\mathrm{Re}}\) \( \newcommand{\ImaginaryPart}{\mathrm{Im}}\)
\( \newcommand{\Argument}{\mathrm{Arg}}\) \( \newcommand{\norm}[1]{\| #1 \|}\)
\( \newcommand{\inner}[2]{\langle #1, #2 \rangle}\)
\( \newcommand{\Span}{\mathrm{span}}\)
\( \newcommand{\id}{\mathrm{id}}\)
\( \newcommand{\Span}{\mathrm{span}}\)
\( \newcommand{\kernel}{\mathrm{null}\,}\)
\( \newcommand{\range}{\mathrm{range}\,}\)
\( \newcommand{\RealPart}{\mathrm{Re}}\)
\( \newcommand{\ImaginaryPart}{\mathrm{Im}}\)
\( \newcommand{\Argument}{\mathrm{Arg}}\)
\( \newcommand{\norm}[1]{\| #1 \|}\)
\( \newcommand{\inner}[2]{\langle #1, #2 \rangle}\)
\( \newcommand{\Span}{\mathrm{span}}\) \( \newcommand{\AA}{\unicode[.8,0]{x212B}}\)
\( \newcommand{\vectorA}[1]{\vec{#1}} % arrow\)
\( \newcommand{\vectorAt}[1]{\vec{\text{#1}}} % arrow\)
\( \newcommand{\vectorB}[1]{\overset { \scriptstyle \rightharpoonup} {\mathbf{#1}} } \)
\( \newcommand{\vectorC}[1]{\textbf{#1}} \)
\( \newcommand{\vectorD}[1]{\overrightarrow{#1}} \)
\( \newcommand{\vectorDt}[1]{\overrightarrow{\text{#1}}} \)
\( \newcommand{\vectE}[1]{\overset{-\!-\!\rightharpoonup}{\vphantom{a}\smash{\mathbf {#1}}}} \)
\( \newcommand{\vecs}[1]{\overset { \scriptstyle \rightharpoonup} {\mathbf{#1}} } \)
\( \newcommand{\vecd}[1]{\overset{-\!-\!\rightharpoonup}{\vphantom{a}\smash {#1}}} \)
\(\newcommand{\avec}{\mathbf a}\) \(\newcommand{\bvec}{\mathbf b}\) \(\newcommand{\cvec}{\mathbf c}\) \(\newcommand{\dvec}{\mathbf d}\) \(\newcommand{\dtil}{\widetilde{\mathbf d}}\) \(\newcommand{\evec}{\mathbf e}\) \(\newcommand{\fvec}{\mathbf f}\) \(\newcommand{\nvec}{\mathbf n}\) \(\newcommand{\pvec}{\mathbf p}\) \(\newcommand{\qvec}{\mathbf q}\) \(\newcommand{\svec}{\mathbf s}\) \(\newcommand{\tvec}{\mathbf t}\) \(\newcommand{\uvec}{\mathbf u}\) \(\newcommand{\vvec}{\mathbf v}\) \(\newcommand{\wvec}{\mathbf w}\) \(\newcommand{\xvec}{\mathbf x}\) \(\newcommand{\yvec}{\mathbf y}\) \(\newcommand{\zvec}{\mathbf z}\) \(\newcommand{\rvec}{\mathbf r}\) \(\newcommand{\mvec}{\mathbf m}\) \(\newcommand{\zerovec}{\mathbf 0}\) \(\newcommand{\onevec}{\mathbf 1}\) \(\newcommand{\real}{\mathbb R}\) \(\newcommand{\twovec}[2]{\left[\begin{array}{r}#1 \\ #2 \end{array}\right]}\) \(\newcommand{\ctwovec}[2]{\left[\begin{array}{c}#1 \\ #2 \end{array}\right]}\) \(\newcommand{\threevec}[3]{\left[\begin{array}{r}#1 \\ #2 \\ #3 \end{array}\right]}\) \(\newcommand{\cthreevec}[3]{\left[\begin{array}{c}#1 \\ #2 \\ #3 \end{array}\right]}\) \(\newcommand{\fourvec}[4]{\left[\begin{array}{r}#1 \\ #2 \\ #3 \\ #4 \end{array}\right]}\) \(\newcommand{\cfourvec}[4]{\left[\begin{array}{c}#1 \\ #2 \\ #3 \\ #4 \end{array}\right]}\) \(\newcommand{\fivevec}[5]{\left[\begin{array}{r}#1 \\ #2 \\ #3 \\ #4 \\ #5 \\ \end{array}\right]}\) \(\newcommand{\cfivevec}[5]{\left[\begin{array}{c}#1 \\ #2 \\ #3 \\ #4 \\ #5 \\ \end{array}\right]}\) \(\newcommand{\mattwo}[4]{\left[\begin{array}{rr}#1 \amp #2 \\ #3 \amp #4 \\ \end{array}\right]}\) \(\newcommand{\laspan}[1]{\text{Span}\{#1\}}\) \(\newcommand{\bcal}{\cal B}\) \(\newcommand{\ccal}{\cal C}\) \(\newcommand{\scal}{\cal S}\) \(\newcommand{\wcal}{\cal W}\) \(\newcommand{\ecal}{\cal E}\) \(\newcommand{\coords}[2]{\left\{#1\right\}_{#2}}\) \(\newcommand{\gray}[1]{\color{gray}{#1}}\) \(\newcommand{\lgray}[1]{\color{lightgray}{#1}}\) \(\newcommand{\rank}{\operatorname{rank}}\) \(\newcommand{\row}{\text{Row}}\) \(\newcommand{\col}{\text{Col}}\) \(\renewcommand{\row}{\text{Row}}\) \(\newcommand{\nul}{\text{Nul}}\) \(\newcommand{\var}{\text{Var}}\) \(\newcommand{\corr}{\text{corr}}\) \(\newcommand{\len}[1]{\left|#1\right|}\) \(\newcommand{\bbar}{\overline{\bvec}}\) \(\newcommand{\bhat}{\widehat{\bvec}}\) \(\newcommand{\bperp}{\bvec^\perp}\) \(\newcommand{\xhat}{\widehat{\xvec}}\) \(\newcommand{\vhat}{\widehat{\vvec}}\) \(\newcommand{\uhat}{\widehat{\uvec}}\) \(\newcommand{\what}{\widehat{\wvec}}\) \(\newcommand{\Sighat}{\widehat{\Sigma}}\) \(\newcommand{\lt}{<}\) \(\newcommand{\gt}{>}\) \(\newcommand{\amp}{&}\) \(\definecolor{fillinmathshade}{gray}{0.9}\)- To understand Enthalpies of Formation and be able to use them to calculate Enthalpies of Reaction
Standard Enthalpies of Formation
A standard enthalpy of formation \(ΔH^\circ_\ce{f}\) is an enthalpy change for a reaction in which exactly 1 mole of a pure substance is formed from free elements in their most stable states under standard state conditions. These values are especially useful for computing or predicting enthalpy changes for chemical reactions that are impractical or dangerous to carry out, or for processes for which it is difficult to make measurements. If we have values for the appropriate standard enthalpies of formation, we can determine the enthalpy change for any reaction, which we will practice in the next section on Hess’s law.
The standard enthalpy of formation of CO2(g) is −393.5 kJ/mol. This is the enthalpy change for the exothermic reaction:
\[\ce{C}(s)+\ce{O2}(g)⟶\ce{CO2}(g)\hspace{20px}ΔH^\circ_\ce{f}=ΔH^\circ_{298}=−393.5\:\ce{kJ} \label{5.4.9}\]
starting with the reactants at a pressure of 1 atm and 25 °C (with the carbon present as graphite, the most stable form of carbon under these conditions) and ending with one mole of CO2, also at 1 atm and 25 °C. For nitrogen dioxide, \(\ce{NO}_{2(g)}\), \(ΔH^\circ_\ce{f}\) is 33.2 kJ/mol. This is the enthalpy change for the reaction:
\[\frac{1}{2}\ce{N2}(g)+\ce{O2}(g)⟶\ce{NO2}(g)\hspace{20px}ΔH^\circ_\ce{f}=ΔH^\circ_{298}=+33.2\: \ce{kJ} \label{5.4.10}\]
A reaction equation with \(\frac{1}{2}\) mole of N2 and 1 mole of O2 is correct in this case because the standard enthalpy of formation always refers to 1 mole of product, NO2(g).
You will find a table of standard enthalpies of formation of many common substances in Tables T1 and T2. These values indicate that formation reactions range from highly exothermic (such as −2984 kJ/mol for the formation of P4O10) to strongly endothermic (such as +226.7 kJ/mol for the formation of acetylene, C2H2). By definition, the standard enthalpy of formation of an element in its most stable form is equal to zero under standard conditions, which is 1 atm for gases and 1 M for solutions.
Definition of Heat of Formation Reactions: https://youtu.be/A20k0CK4doI
Ozone, O3(g), forms from oxygen, O2(g), by an endothermic process. Ultraviolet radiation is the source of the energy that drives this reaction in the upper atmosphere. Assuming that both the reactants and products of the reaction are in their standard states, determine the standard enthalpy of formation, \(ΔH^\circ_\ce{f}\) of ozone from the following information:
Solution \(ΔH^\circ_\ce{f}\) is the enthalpy change for the formation of one mole of a substance in its standard state from the elements in their standard states. Thus, \(ΔH^\circ_\ce{f}\) for O3(g) is the enthalpy change for the reaction:
\[\dfrac{3}{2}\ce{O2}(g)⟶\ce{O3}(g) \nonumber\]
For the formation of 2 mol of O3(g), \(ΔH^\circ_{298}=+286\: \ce{kJ}\). This ratio, \(\mathrm{\left(\dfrac{286\:kJ}{2\:mol\:O_3}\right)}\), can be used as a conversion factor to find the heat produced when 1 mole of O3(g) is formed, which is the enthalpy of formation for O3(g):
Therefore, \(ΔH^\circ_\ce{f}[\ce{O3}(g)]=\ce{+143\: kJ/mol}\).
Hydrogen gas, H2, reacts explosively with gaseous chlorine, Cl2, to form hydrogen chloride, HCl(g). What is the enthalpy change for the reaction of 1 mole of H2(g) with 1 mole of Cl2(g) if both the reactants and products are at standard state conditions? The standard enthalpy of formation of HCl(g) is −92.3 kJ/mol.
- Answer
-
For the reaction
\[\ce{H2}(g)+\ce{Cl2}(g)⟶\ce{2HCl}(g)\hspace{20px}ΔH^\circ_{298}=\mathrm{−184.6\:kJ} \nonumber\]
Write the heat of formation reaction equations for:
- \(\ce{C2H_5OH}_{(l)}\)
- \(\ce{Ca_3(PO_4)}_{2(s)}\)
Solution
Remembering that \(ΔH^\circ_\ce{f}\) reaction equations are for forming 1 mole of the compound from its constituent elements under standard conditions, we have:
- \(\ce{2C}(s,\:\ce{graphite})+\ce{3H2}(g)+\frac{1}{2}\ce{O2}(g)⟶\ce{C2H5OH}(l)\)
- \(\ce{3Ca}(s)+\frac{1}{2}\ce{P4}(s)+\ce{4O2}(g)⟶\ce{Ca3(PO4)2}(s)\)
Note: The standard state of carbon is graphite, and phosphorus exists as \(P_4\).
Write the heat of formation reaction equations for:
- \(\ce{C_2H_5OC_2H}_{5(l)}\)
- \(\ce{Na_2CO}_{3(s)}\)
- Answer a
-
\(\ce{4C}(s,\:\ce{graphite})+\ce{5H2}(g)+\frac{1}{2}\ce{O2}(g)⟶\ce{C2H5OC2H5}(l)\);
- Answer b
-
\(\ce{2Na}(s)+\ce{C}(s,\:\ce{graphite})+\dfrac{3}{2}\ce{O2}(g)⟶\ce{Na2CO3}(s)\)
Calculating DH° using DHf°: https://youtu.be/Y3aJJno9W2c
Standard Enthalpies of Reaction
Tabulated values of standard enthalpies of formation can be used to calculate enthalpy changes for any reaction involving substances whose \(\Delta{H_f^o}\) values are known. The standard enthalpy of reaction \(\Delta{H_{rxn}^o}\) is the enthalpy change that occurs when a reaction is carried out with all reactants and products in their standard states. Consider the general reaction
\[ aA + bB \rightarrow cC + dD \label{7.8.3}\]
where \(A\), \(B\), \(C\), and \(D\) are chemical substances and \(a\), \(b\), \(c\), and \(d\) are their stoichiometric coefficients. The magnitude of \(ΔH^ο\) is the sum of the standard enthalpies of formation of the products, each multiplied by its appropriate coefficient, minus the sum of the standard enthalpies of formation of the reactants, also multiplied by their coefficients:
\[ \Delta H_{rxn}^{o} = \underbrace{ \left [c\Delta H_{f}^{o}\left ( C \right ) + d\Delta H_{f}^{o}\left ( D \right ) \right ] }_{\text{products} } - \underbrace{ \left [a\Delta H_{f}^{o}\left ( A \right ) + b\Delta H_{f}^{o}\left ( B \right ) \right ]}_{\text{reactants }} \label{7.8.4} \]
More generally, we can write
\[ \Delta H_{rxn}^{o} = \sum m\Delta H_{f}^{o}\left ( products \right ) - \sum n\Delta H_{f}^{o}\left ( reactants \right ) \label{7.8.5} \]
where the symbol \(\sum\) means “sum of” and \(m\) and \(n\) are the stoichiometric coefficients of each of the products and the reactants, respectively. “Products minus reactants” summations such as Equation \(\ref{7.8.5}\) arise from the fact that enthalpy is a state function. Because many other thermochemical quantities are also state functions, “products minus reactants” summations are very common in chemistry; we will encounter many others in subsequent chapters.
"Products minus reactants" summations are typical of state functions.
To demonstrate the use of tabulated ΔHο values, we will use them to calculate \(ΔH_{rxn}\) for the combustion of glucose, the reaction that provides energy for your brain:
\[ \ce{ C6H12O6 (s) + 6O2 (g) \rightarrow 6CO2 (g) + 6H2O (l)} \label{7.8.6} \]
Using Equation \(\ref{7.8.5}\), we write
\[ \Delta H_{f}^{o} =\left \{ 6\Delta H_{f}^{o}\left [ CO_{2}\left ( g \right ) \right ] + 6\Delta H_{f}^{o}\left [ H_{2}O\left ( g \right ) \right ] \right \} - \left \{ \Delta H_{f}^{o}\left [ C_{6}H_{12}O_{6}\left ( s \right ) \right ] + 6\Delta H_{f}^{o}\left [ O_{2}\left ( g \right ) \right ] \right \} \label{7.8.7} \]
From Table T1, the relevant ΔHοf values are ΔHοf [CO2(g)] = -393.5 kJ/mol, ΔHοf [H2O(l)] = -285.8 kJ/mol, and ΔHοf [C6H12O6(s)] = -1273.3 kJ/mol. Because O2(g) is a pure element in its standard state, ΔHοf [O2(g)] = 0 kJ/mol. Inserting these values into Equation \(\ref{7.8.7}\) and changing the subscript to indicate that this is a combustion reaction, we obtain
\[ \begin{align} \Delta H_{comb}^{o} &= \left [ 6\left ( -393.5 \; kJ/mol \right ) + 6 \left ( -285.8 \; kJ/mol \right ) \right ] - \left [-1273.3 + 6\left ( 0 \; kJ\;mol \right ) \right ] \label{7.8.8} \\[4pt] &= -2802.5 \; kJ/mol \end{align} \]
As illustrated in Figure \(\PageIndex{2}\), we can use Equation \(\ref{7.8.8}\) to calculate \(ΔH^ο_f\) for glucose because enthalpy is a state function. The figure shows two pathways from reactants (middle left) to products (bottom). The more direct pathway is the downward green arrow labeled \(ΔH^ο_{comb}\). The alternative hypothetical pathway consists of four separate reactions that convert the reactants to the elements in their standard states (upward purple arrow at left) and then convert the elements into the desired products (downward purple arrows at right). The reactions that convert the reactants to the elements are the reverse of the equations that define the \(ΔH^ο_f\) values of the reactants. Consequently, the enthalpy changes are
\[ \begin{align} \Delta H_{1}^{o} &= \Delta H_{f}^{o} \left [ glucose \left ( s \right ) \right ] \nonumber \\[4pt] &= -1 \; \cancel{mol \; glucose}\left ( \dfrac{1273.3 \; kJ}{1 \; \cancel{mol \; glucose}} \right ) \nonumber \\[4pt] &= +1273.3 \; kJ \nonumber \\[4pt] \Delta H_{2}^{o} &= 6 \Delta H_{f}^{o} \left [ O_{2} \left ( g \right ) \right ] \nonumber \\[4pt] & =6 \; \cancel{mol \; O_{2}}\left ( \dfrac{0 \; kJ}{1 \; \cancel{mol \; O_{2}}} \right ) \nonumber \\[4pt] &= 0 \; kJ \end{align} \label{7.8.9} \]
Recall that when we reverse a reaction, we must also reverse the sign of the accompanying enthalpy change (Equation \ref{7.8.4} since the products are now reactants and vice versa.
The overall enthalpy change for conversion of the reactants (1 mol of glucose and 6 mol of O2) to the elements is therefore +1273.3 kJ.

The reactions that convert the elements to final products (downward purple arrows in Figure \(\PageIndex{2}\)) are identical to those used to define the ΔHοf values of the products. Consequently, the enthalpy changes (from Table T1) are
\[ \begin{matrix} \Delta H_{3}^{o} = \Delta H_{f}^{o} \left [ CO_{2} \left ( g \right ) \right ] = 6 \; \cancel{mol \; CO_{2}}\left ( \dfrac{393.5 \; kJ}{1 \; \cancel{mol \; CO_{2}}} \right ) = -2361.0 \; kJ \\ \Delta H_{4}^{o} = 6 \Delta H_{f}^{o} \left [ H_{2}O \left ( l \right ) \right ] = 6 \; \cancel{mol \; H_{2}O}\left ( \dfrac{-285.8 \; kJ}{1 \; \cancel{mol \; H_{2}O}} \right ) = -1714.8 \; kJ \end{matrix} \]
The overall enthalpy change for the conversion of the elements to products (6 mol of carbon dioxide and 6 mol of liquid water) is therefore −4075.8 kJ. Because enthalpy is a state function, the difference in enthalpy between an initial state and a final state can be computed using any pathway that connects the two. Thus the enthalpy change for the combustion of glucose to carbon dioxide and water is the sum of the enthalpy changes for the conversion of glucose and oxygen to the elements (+1273.3 kJ) and for the conversion of the elements to carbon dioxide and water (−4075.8 kJ):
\[ \Delta H_{comb}^{o} = +1273.3 \; kJ +\left ( -4075.8 \; kJ \right ) = -2802.5 \; kJ \label{7.8.10} \]
This is the same result we obtained using the “products minus reactants” rule (Equation \(\ref{7.8.5}\)) and ΔHοf values. The two results must be the same because Equation \(\ref{7.8.10}\) is just a more compact way of describing the thermochemical cycle shown in Figure \(\PageIndex{1}\).
Long-chain fatty acids such as palmitic acid (\(\ce{CH3(CH2)14CO2H}\)) are one of the two major sources of energy in our diet (\(ΔH^o_f\) =−891.5 kJ/mol). Use the data in Table T1 to calculate ΔHοcomb for the combustion of palmitic acid. Based on the energy released in combustion per gram, which is the better fuel — glucose or palmitic acid?
Given: compound and \(ΔH^ο_{f}\) values
Asked for: \(ΔH^ο_{comb}\) per mole and per gram
Strategy:
- After writing the balanced chemical equation for the reaction, use Equation \(\ref{7.8.5}\) and the values from Table T1 to calculate \(ΔH^ο_{comb}\) the energy released by the combustion of 1 mol of palmitic acid.
- Divide this value by the molar mass of palmitic acid to find the energy released from the combustion of 1 g of palmitic acid. Compare this value with the value calculated in Equation \(\ref{7.8.8}\) for the combustion of glucose to determine which is the better fuel.
Solution:
A To determine the energy released by the combustion of palmitic acid, we need to calculate its \(ΔH^ο_f\). As always, the first requirement is a balanced chemical equation:
\[C_{16}H_{32}O_{2(s)} + 23O_{2(g)} \rightarrow 16CO_{2(g)} + 16H_2O_{(l)} \nonumber \]
Using Equation \(\ref{7.8.5}\) (“products minus reactants”) with ΔHοf values from Table T1 (and omitting the physical states of the reactants and products to save space) gives
\[ \begin{align*} \Delta H_{comb}^{o} &= \sum m \Delta H^o_f\left( {products} \right) - \sum n \Delta H^o_f \left( {reactants} \right) \\[4pt] &= \left [ 16\left ( -393.5 \; kJ/mol \; CO_{2} \right ) + 16\left ( -285.8 \; kJ/mol \; H_{2}O \; \right ) \right ] \\[4pt] & - \left [ -891.5 \; kJ/mol \; C_{16}H_{32}O_{2} + 23\left ( 0 \; kJ/mol \; O_{2} \; \right ) \right ] \\[4pt] &= -9977.3 \; kJ/mol \nonumber \end{align*} \]
This is the energy released by the combustion of 1 mol of palmitic acid.
B The energy released by the combustion of 1 g of palmitic acid is
\( \Delta H_{comb}^{o} \; per \; gram =\left ( \dfrac{9977.3 \; kJ}{\cancel{1 \; mol}} \right ) \left ( \dfrac{\cancel{1 \; mol}}{256.42 \; g} \right )= -38.910 \; kJ/g \nonumber \)
As calculated in Equation \(\ref{7.8.8}\), \(ΔH^o_f\) of glucose is −2802.5 kJ/mol. The energy released by the combustion of 1 g of glucose is therefore
\( \Delta H_{comb}^{o} \; per \; gram =\left ( \dfrac{-2802.5 \; kJ}{\cancel{1\; mol}} \right ) \left ( \dfrac{\cancel{1 \; mol}}{180.16\; g} \right ) = -15.556 \; kJ/g \nonumber \)
The combustion of fats such as palmitic acid releases more than twice as much energy per gram as the combustion of sugars such as glucose. This is one reason many people try to minimize the fat content in their diets to lose weight.
Use Table T1 to calculate \(ΔH^o_{rxn}\) for the water–gas shift reaction, which is used industrially on an enormous scale to obtain H2(g):
\[ \ce{ CO ( g ) + H2O (g ) -> CO2 (g) + H2 ( g )} \nonumber\]
- Answer
-
−41.2 kJ/mol
We can also measure the enthalpy change for another reaction, such as a combustion reaction, and then use it to calculate a compound’s \(ΔH^ο_f\) which we cannot obtain otherwise. This procedure is illustrated in Example \(\PageIndex{3}\).
Beginning in 1923, tetraethyllead [\(\ce{(C2H5)4Pb}\)] was used as an antiknock additive in gasoline in the United States. Its use was completely phased out in 1986 because of the health risks associated with chronic lead exposure. Tetraethyllead is a highly poisonous, colorless liquid that burns in air to give an orange flame with a green halo. The combustion products are \(\ce{CO2(g)}\), \(\ce{H2O(l)}\), and red \(\ce{PbO(s)}\). What is the standard enthalpy of formation of tetraethyllead, given that \(ΔH^ο_f\) is −19.29 kJ/g for the combustion of tetraethyllead and \(ΔH^ο_f\) of red PbO(s) is −219.0 kJ/mol?
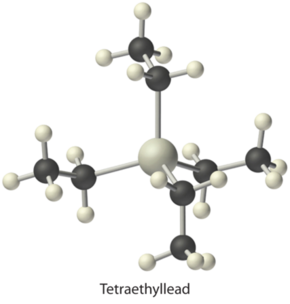
Given: reactant, products, and \(ΔH^ο_{comb}\) values
Asked for: \(ΔH^ο_f\) of the reactants
Strategy:
- Write the balanced chemical equation for the combustion of tetraethyl lead. Then insert the appropriate quantities into Equation \(\ref{7.8.5}\) to get the equation for ΔHοf of tetraethyl lead.
- Convert \(ΔH^ο_{comb}\) per gram given in the problem to \(ΔH^ο_{comb}\) per mole by multiplying \(ΔH^ο_{comb}\) per gram by the molar mass of tetraethyllead.
- Use Table T1 to obtain values of \(ΔH^ο_f\) for the other reactants and products. Insert these values into the equation for \(ΔH^ο_f\) of tetraethyl lead and solve the equation.
Solution:
A The balanced chemical equation for the combustion reaction is as follows:
\[\ce{2(C2H5)4Pb(l) + 27O2(g) → 2PbO(s) + 16CO2(g) + 20H2O(l)} \nonumber\]
Using Equation \(\ref{7.8.5}\) gives
\[ \Delta H_{comb}^{o} = \left [ 2 \Delta H_{f}^{o}\left ( PbO \right ) + 16 \Delta H_{f}^{o}\left ( CO_{2} \right ) + 20 \Delta H_{f}^{o}\left ( H_{2}O \right )\right ] - \left [2 \Delta H_{f}^{o}\left ( \left ( C_{2}H_{5} \right ) _{4} Pb \right ) + 27 \Delta H_{f}^{o}\left ( O_{2} \right ) \right ] \nonumber \]
Solving for \(ΔH^o_f [\ce{(C2H5)4Pb}]\) gives
\[ \Delta H_{f}^{o}\left ( \left ( C_{2}H_{5} \right ) _{4} Pb \right ) = \Delta H_{f}^{o}\left ( PbO \right ) + 8 \Delta H_{f}^{o}\left ( CO_{2} \right ) + 10 \Delta H_{f}^{o}\left ( H_{2}O \right ) - \dfrac{27}{2} \Delta H_{f}^{o}\left ( O_{2} \right ) - \dfrac{\Delta H_{comb}^{o}}{2} \nonumber \]
The values of all terms other than \(ΔH^o_f [\ce{(C2H5)4Pb}]\) are given in Table T1.
B The magnitude of \(ΔH^o_{comb}\) is given in the problem in kilojoules per gram of tetraethyl lead. We must therefore multiply this value by the molar mass of tetraethyl lead (323.44 g/mol) to get \(ΔH^o_{comb}\) for 1 mol of tetraethyl lead:
\[\begin{align*} \Delta H_{comb}^{o} &= \left ( \dfrac{-19.29 \; kJ}{\cancel{g}} \right )\left ( \dfrac{323.44 \; \cancel{g}}{mol} \right ) \\[4pt] &= -6329 \; kJ/mol \end{align*} \]
Because the balanced chemical equation contains 2 mol of tetraethyllead, \(ΔH^o_{rxn}\) is
\[\begin{align*} \Delta H_{rxn}^{o} &= 2 \; \cancel{mol \; \left ( C_{2}H{5}\right )_4 Pb} \left ( \dfrac{-6329 \; kJ}{1 \; \cancel{mol \; \left ( C_{2}H{5}\right )_4 Pb }} \right ) \\[4pt] &= -12,480 \; kJ \end{align*}\]
C Inserting the appropriate values into the equation for \(ΔH^o_f [\ce{(C2H5)4Pb}]\) gives
\[ \begin{align*} \Delta H_{f}^{o} \left [ \left (C_{2}H_{4} \right )_{4}Pb \right ] & = \left [1 \; mol \;PbO \;\times 219.0 \;kJ/mol \right ]+\left [8 \; mol \;CO_{2} \times \left (-393.5 \; kJ/mol \right )\right ] +\left [10 \; mol \; H_{2}O \times \left ( -285.8 \; kJ/mol \right )\right ] + \left [-27/2 \; mol \; O_{2}) \times 0 \; kJ/mol \; O_{2}\right ] \left [12,480.2 \; kJ/mol \; \left ( C_{2}H_{5} \right )_{4}Pb \right ]\\[4pt]
&= -219.0 \; kJ -3148 \; kJ - 2858 kJ - 0 kJ + 6240 \; kJ = 15 kJ/mol \end{align*}\]
Ammonium sulfate, \(\ce{(NH4)2SO4}\), is used as a fire retardant and wood preservative; it is prepared industrially by the highly exothermic reaction of gaseous ammonia with sulfuric acid:
\[ \ce{2NH3(g) + H2SO4(aq) \rightarrow (NH4)2SO4(s)} \nonumber \]
The value of \(ΔH^o_{rxn}\) is -179.4 kJ/mole \(\ce{H2SO4}\). Use the data in Table T1 to calculate the standard enthalpy of formation of ammonium sulfate (in kilojoules per mole).
- Answer
-
−1181 kJ/mol
Calculate the heat of combustion of 1 mole of ethanol, C2H5OH(l), when H2O(l) and CO2(g) are formed. Use the following enthalpies of formation: C2H5OH(l), −278 kJ/mol; H2O(l), −286 kJ/mol; and CO2(g), −394 kJ/mol.
- Answer
-
−1368 kJ/mol
Summary
The standard enthalpy of formation, \(ΔH^\circ_\ce{f}\), is the enthalpy change accompanying the formation of 1 mole of a substance from the elements in their most stable states at 1 bar (standard state). Many of the processes are carried out at 298.15 K.
Key Equations
- \(ΔU=q+w\)
- \(ΔH^\circ_\ce{reaction}=∑n×ΔH^\circ_\ce{f}\ce{(products)}−∑n×ΔH^\circ_\ce{f}\ce{(reactants)}\)
Footnotes
- 1 For more on algal fuel, see www.theguardian.com/environme...n-fuel-problem.
Glossary
- standard enthalpy of combustion (\(ΔH^\circ_\ce{c}\))
- heat released when one mole of a compound undergoes complete combustion under standard conditions
- standard enthalpy of formation (\(ΔH^\circ_\ce{f}\))
- enthalpy change of a chemical reaction in which 1 mole of a pure substance is formed from its elements in their most stable states under standard state conditions
- standard state
- set of physical conditions as accepted as common reference conditions for reporting thermodynamic properties; 1 bar of pressure, and solutions at 1 molar concentrations, usually at a temperature of 298.15 K