7.1: Why and how CW EPR spectroscopy is done
- Page ID
- 370942
\( \newcommand{\vecs}[1]{\overset { \scriptstyle \rightharpoonup} {\mathbf{#1}} } \)
\( \newcommand{\vecd}[1]{\overset{-\!-\!\rightharpoonup}{\vphantom{a}\smash {#1}}} \)
\( \newcommand{\id}{\mathrm{id}}\) \( \newcommand{\Span}{\mathrm{span}}\)
( \newcommand{\kernel}{\mathrm{null}\,}\) \( \newcommand{\range}{\mathrm{range}\,}\)
\( \newcommand{\RealPart}{\mathrm{Re}}\) \( \newcommand{\ImaginaryPart}{\mathrm{Im}}\)
\( \newcommand{\Argument}{\mathrm{Arg}}\) \( \newcommand{\norm}[1]{\| #1 \|}\)
\( \newcommand{\inner}[2]{\langle #1, #2 \rangle}\)
\( \newcommand{\Span}{\mathrm{span}}\)
\( \newcommand{\id}{\mathrm{id}}\)
\( \newcommand{\Span}{\mathrm{span}}\)
\( \newcommand{\kernel}{\mathrm{null}\,}\)
\( \newcommand{\range}{\mathrm{range}\,}\)
\( \newcommand{\RealPart}{\mathrm{Re}}\)
\( \newcommand{\ImaginaryPart}{\mathrm{Im}}\)
\( \newcommand{\Argument}{\mathrm{Arg}}\)
\( \newcommand{\norm}[1]{\| #1 \|}\)
\( \newcommand{\inner}[2]{\langle #1, #2 \rangle}\)
\( \newcommand{\Span}{\mathrm{span}}\) \( \newcommand{\AA}{\unicode[.8,0]{x212B}}\)
\( \newcommand{\vectorA}[1]{\vec{#1}} % arrow\)
\( \newcommand{\vectorAt}[1]{\vec{\text{#1}}} % arrow\)
\( \newcommand{\vectorB}[1]{\overset { \scriptstyle \rightharpoonup} {\mathbf{#1}} } \)
\( \newcommand{\vectorC}[1]{\textbf{#1}} \)
\( \newcommand{\vectorD}[1]{\overrightarrow{#1}} \)
\( \newcommand{\vectorDt}[1]{\overrightarrow{\text{#1}}} \)
\( \newcommand{\vectE}[1]{\overset{-\!-\!\rightharpoonup}{\vphantom{a}\smash{\mathbf {#1}}}} \)
\( \newcommand{\vecs}[1]{\overset { \scriptstyle \rightharpoonup} {\mathbf{#1}} } \)
\( \newcommand{\vecd}[1]{\overset{-\!-\!\rightharpoonup}{\vphantom{a}\smash {#1}}} \)
\(\newcommand{\avec}{\mathbf a}\) \(\newcommand{\bvec}{\mathbf b}\) \(\newcommand{\cvec}{\mathbf c}\) \(\newcommand{\dvec}{\mathbf d}\) \(\newcommand{\dtil}{\widetilde{\mathbf d}}\) \(\newcommand{\evec}{\mathbf e}\) \(\newcommand{\fvec}{\mathbf f}\) \(\newcommand{\nvec}{\mathbf n}\) \(\newcommand{\pvec}{\mathbf p}\) \(\newcommand{\qvec}{\mathbf q}\) \(\newcommand{\svec}{\mathbf s}\) \(\newcommand{\tvec}{\mathbf t}\) \(\newcommand{\uvec}{\mathbf u}\) \(\newcommand{\vvec}{\mathbf v}\) \(\newcommand{\wvec}{\mathbf w}\) \(\newcommand{\xvec}{\mathbf x}\) \(\newcommand{\yvec}{\mathbf y}\) \(\newcommand{\zvec}{\mathbf z}\) \(\newcommand{\rvec}{\mathbf r}\) \(\newcommand{\mvec}{\mathbf m}\) \(\newcommand{\zerovec}{\mathbf 0}\) \(\newcommand{\onevec}{\mathbf 1}\) \(\newcommand{\real}{\mathbb R}\) \(\newcommand{\twovec}[2]{\left[\begin{array}{r}#1 \\ #2 \end{array}\right]}\) \(\newcommand{\ctwovec}[2]{\left[\begin{array}{c}#1 \\ #2 \end{array}\right]}\) \(\newcommand{\threevec}[3]{\left[\begin{array}{r}#1 \\ #2 \\ #3 \end{array}\right]}\) \(\newcommand{\cthreevec}[3]{\left[\begin{array}{c}#1 \\ #2 \\ #3 \end{array}\right]}\) \(\newcommand{\fourvec}[4]{\left[\begin{array}{r}#1 \\ #2 \\ #3 \\ #4 \end{array}\right]}\) \(\newcommand{\cfourvec}[4]{\left[\begin{array}{c}#1 \\ #2 \\ #3 \\ #4 \end{array}\right]}\) \(\newcommand{\fivevec}[5]{\left[\begin{array}{r}#1 \\ #2 \\ #3 \\ #4 \\ #5 \\ \end{array}\right]}\) \(\newcommand{\cfivevec}[5]{\left[\begin{array}{c}#1 \\ #2 \\ #3 \\ #4 \\ #5 \\ \end{array}\right]}\) \(\newcommand{\mattwo}[4]{\left[\begin{array}{rr}#1 \amp #2 \\ #3 \amp #4 \\ \end{array}\right]}\) \(\newcommand{\laspan}[1]{\text{Span}\{#1\}}\) \(\newcommand{\bcal}{\cal B}\) \(\newcommand{\ccal}{\cal C}\) \(\newcommand{\scal}{\cal S}\) \(\newcommand{\wcal}{\cal W}\) \(\newcommand{\ecal}{\cal E}\) \(\newcommand{\coords}[2]{\left\{#1\right\}_{#2}}\) \(\newcommand{\gray}[1]{\color{gray}{#1}}\) \(\newcommand{\lgray}[1]{\color{lightgray}{#1}}\) \(\newcommand{\rank}{\operatorname{rank}}\) \(\newcommand{\row}{\text{Row}}\) \(\newcommand{\col}{\text{Col}}\) \(\renewcommand{\row}{\text{Row}}\) \(\newcommand{\nul}{\text{Nul}}\) \(\newcommand{\var}{\text{Var}}\) \(\newcommand{\corr}{\text{corr}}\) \(\newcommand{\len}[1]{\left|#1\right|}\) \(\newcommand{\bbar}{\overline{\bvec}}\) \(\newcommand{\bhat}{\widehat{\bvec}}\) \(\newcommand{\bperp}{\bvec^\perp}\) \(\newcommand{\xhat}{\widehat{\xvec}}\) \(\newcommand{\vhat}{\widehat{\vvec}}\) \(\newcommand{\uhat}{\widehat{\uvec}}\) \(\newcommand{\what}{\widehat{\wvec}}\) \(\newcommand{\Sighat}{\widehat{\Sigma}}\) \(\newcommand{\lt}{<}\) \(\newcommand{\gt}{>}\) \(\newcommand{\amp}{&}\) \(\definecolor{fillinmathshade}{gray}{0.9}\)Sensitivity advantages of CW EPR spectroscopy
In NMR spectroscopy, CW techniques have been almost completely displaced by Fourier transform (FT) techniques, except for a few niche applications. FT techniques have a sensitivity advantage if the spectrum contains large sections of baseline and the whole spectrum can be excited simultaneously by the pulses. Neither condition is usually fulfilled in EPR spectroscopy. For two reasons, FT techniques lose sensitivity in EPR spectroscopy compared to the CW experiment. First, while typical NMR spectra comfortably fit into the bandwidth of a welldesigned critically coupled radiofrequency resonance circuit, EPR spectra are much broader than the bandwidth of a microwave resonator with high quality factor. Broadening detection bandwidth and proportionally lowering the quality factor \(Q\) of the resonator reduces signal-to-noise ratio unless the absorption lineshape is infinitely broad. A quality factor of the order of 10 ’ 000 , which can be achieved with cavity resonators, corresponds to a bandwidth of roughly \(1 \mathrm{MHz}\) at X-band frequencies around \(9.6 \mathrm{GHz}\). The intrinsic high sensitivity of detection in such a narrow band can be used only in a CW experiment. Second, even if the resonator is overcoupled to a much lower quality factor or resonators with intrinsically lower \(Q\) are used (the sensitivity loss can partially be compensated by a higher filling factor of such resonators), residual power from a high-power microwave pulse requires about \(100 \mathrm{~ns}\) in order to decay below the level of an EPR signal. This dead time is often a significant fraction of the transverse relaxation time of electron spins, which entails signal loss by relaxation. In contrast, in NMR spectroscopy the dead time is usually negligibly short compared to relaxation times. In many cases, the dead time in pulsed EPR spectroscopy even strongly exceeds \(T_{2}\). In this situation FT EPR is impossible, even with echo refocusing, while CW EPR spectra can still be measured. This case usually applies to transition metal complexes at room temperature and to many rare earth metal complexes and high-spin Fe(III) complexes even down to the boiling point of liquid helium at normal pressure (4.2 K). For these reasons, any unknown potentially paramagnetic sample should first be characterized by CW EPR spectroscopy. Pulsed EPR techniques are required if the resolution of CW EPR spectroscopy provides insufficient information to assign a structure. This applies mainly to small hyperfine couplings in organic radicals and of ligand nuclei in transition metal complexes (see Chapter 8) and to the measurement of distances between electron spins in the nanometer range (see Chapter 9). At temperatures where pulse EPR signals can be obtained, measurement of relaxation times is also easier and more precise with pulsed EPR techniques.
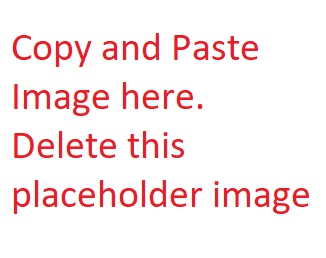
The CW EPR experiment
Since the bandwidth of an optimized microwave resonator is much smaller than the typical width of EPR spectra, it is impractical to sweep the frequency at constant magnetic field in order to obtain a spectrum. Instead, the microwave frequency is kept constant and coincides with the resonator frequency at all times. The resonance condition for the spins is established by sweeping the magnetic field \(B_{0}\). Another difficulty arises from the weak magnetic coupling of the spins to the exciting electromagnetic field. Only a very small fraction of the excitation power is therefore observed. This problem is solved as follows. First, direct transmission of excitation power to the detector is prevented by a circulator (Figure 7.1). Power that enters port 1 can only leave to the sample through port 2 . Power that comes from the sample through port 2 can only leave to the detector diode through port 3. Second, the resonator is critically coupled. This means that all microwave power coming from the source that is incident to the resonator enters the resonator and is converted to heat by the impedance (complex resistance) of the resonator. If the sample is off resonant and thus does not absorb microwave, no microwave power leaves the resonator through port 3. If now the magnetic field \(B_{0}\) is set to the resonance condition and the sample resonantly absorbs microwave, this means that the impedance of resonator + sample has changed. The resonator is no longer critically coupled and some of the incoming microwave is reflected. This microwave leaves the circulator through port 3 and is incident on the detector diode.
This reflected power at resonance absorption can be very weak at low sample concentration. It is therefore important to detect it sensitively. A microwave diode is only weakly sensitive to a change in incident power at low power (Fig. 7.2, input voltage is proportional to the square root of power). The diode is most sensitive to amplitude changes near its operating point, marked green in Fig. 7.2. Hence, the diode must be biased to its operating point by adding constant power from a reference arm. The phase of the reference arm must be adjusted so that microwave coming from the resonator and microwave coming from the reference arm interfere constructively.
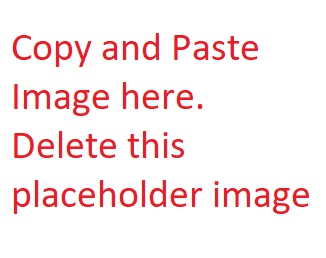
A further problem arises from the fact that microwave diodes are broadband detectors. On the one hand, this is useful, since samples can significantly shift resonator frequency. On the other hand, broadband detectors also collect noise from a broad frequency band. This decreases signal-to-noise ratio and must be countered by limiting the detection bandwidth to the signal bandwidth or even below. Such bandwidth limitation can be realized most easily by effect modulation and phase sensitive detection. By applying a small sinusoidal magnetic field modulation with typical frequency of \(100 \mathrm{kHz}\) and typical amplitude of \(0.01-1 \mathrm{mT}\), the signal component at detector diode output becomes modulated with the same frequency, whereas noise is uncorrelated to the modulation. Demodulation with a reference signal from the field modulation generator (Figure 7.1) by a phase-sensitive detector amplifies the signal and limits bandwidth to the modulation frequency.
Effect modulation with phase-sensitive detection measures the derivative of the absorption lineshape, as long as the modulation amplitude \(\Delta B_{0}\) is much smaller than the width of the EPR line (Fig. 7.3). Since signal-to-noise ratio is proportional to \(\Delta B_{0}\), one usually measures at \(\Delta B_{0} \approx \Delta B_{\mathrm{pp}} / 3\), where lineshape distortion is tolerable for almost all applications. Precise lineshape analysis may require \(\Delta B_{0} \leq \Delta B_{\mathrm{pp}} / 5\), whereas maximum sensitivity at the expense of significant artificial line broadening is obtained at \(\Delta B_{0}=\Delta B_{\mathrm{pp}}\). The modulation frequency should not be broader than the linewidth in frequency units. However, with the standard modulation frequency of \(100 \mathrm{kHz}\) that corresponds on a magnetic field scale to only \(3.6 \mu \mathrm{T}\) at \(g=g_{e}\), this is rarely a problem.
Considerations on sample preparation
Since electron spins have a much larger magnetic moment than nuclear spins, electron-electron couplings lead to significant line broadening in concentrated solutions. Concentrations of paramagnetic centers should not usually exceed \(1 \mathrm{mM}\) in order to avoid such broadening. For organic radicals in liquid solution it may be necessary to dilute the sample to \(100 \mu \mathrm{M}\) in order to achieve ultimate resolution. For paramagnetic metal dopants in diamagnetic host compounds, at most \(1 \%\) of the diamagnetic sites should be substituted by paramagnetic centers. Such concentrations can be detected easily and with good signal-to-noise ratio. For most samples, good spectra can be obtained down to the \(1 \mu \mathrm{M}\) range in solution and down to the 100 ppm dopant range in solids.
Line broadening in liquid solution can also arise from diffusional collision of paramagnetic
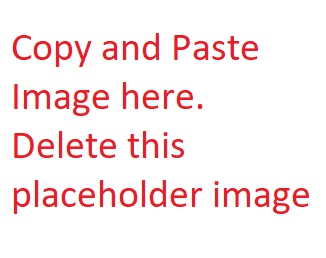
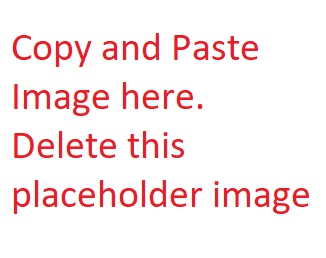
species with paramagnetic triplet oxygen (Figure 7.4). During such a collision, wavefunctions of the two molecules overlap and, since electrons are undistinguishable particles, spin states of all unpaired electrons in both molecules are arbitrarily redistributed when the two molecules separate again. The stochastic diffusional encounters thus lead to additional flips of the observed electron spins, which corresponds to relaxation and shortens longitudinal relaxation time \(T_{1}\). Since the linewidth is proportional to \(T_{2}\) and \(T_{2}\) cannot be longer than \(2 T_{1}\), frequent collisional encounters of paramagnetic species lead to line broadening. Such line broadening increases with decreasing viscosity (faster diffusion) and increasing oxygen concentration. The effect is stronger in apolar solvents, where oxygen solubility is higher than in polar solvents, but it is often significant even in aqueous solution. Best resolution is obtained if the sample is free of oxygen. The same mechanism leads to line broadening at high concentration of a paramagnetic species in liquid solution. In the solid state, line broadening at high concentration is mainly due to dipole-dipole coupling. Often, the anisotropically broadened EPR spectrum in the solid state is of interest, as it provides information on \(g\) anisotropy and anisotropic hyperfine couplings. This may require freezing of a solution of the species of interest. Usually, the species will precipitate if the solvent crystallizes, which may cause line broadening and, in extreme cases, even collapse of the hyperfine structure and averaging of \(g\) anisotropy by exchange between neighboring paramagnetic species. These problems are prevented if the solvent forms a glass, as is often the case for solvents that have methyl groups or can form hydrogen bonds in very different geometries. Typical glass-forming solvents are toluene, 2-methyltetrahydrofuran, ethanol, ethylene glycol, and glycerol. Aqueous solutions require addition of at least \(25 \%\) glycerol as a cryoprotectant. In most cases, crystallization will still occur on slow cooling. Samples are therefore shock frozen by immersion of the sample tube into liquid nitrogen. Glass tubes would break on direct immersion into liquid nitrogen, but EPR spectra have to be measured in fused silica sample tubes anyway, since glass invariably contains a detectable amount of paramagnetic iron impurities.