1.1: The Origins of the Universe
- Page ID
- 240487
\( \newcommand{\vecs}[1]{\overset { \scriptstyle \rightharpoonup} {\mathbf{#1}} } \)
\( \newcommand{\vecd}[1]{\overset{-\!-\!\rightharpoonup}{\vphantom{a}\smash {#1}}} \)
\( \newcommand{\id}{\mathrm{id}}\) \( \newcommand{\Span}{\mathrm{span}}\)
( \newcommand{\kernel}{\mathrm{null}\,}\) \( \newcommand{\range}{\mathrm{range}\,}\)
\( \newcommand{\RealPart}{\mathrm{Re}}\) \( \newcommand{\ImaginaryPart}{\mathrm{Im}}\)
\( \newcommand{\Argument}{\mathrm{Arg}}\) \( \newcommand{\norm}[1]{\| #1 \|}\)
\( \newcommand{\inner}[2]{\langle #1, #2 \rangle}\)
\( \newcommand{\Span}{\mathrm{span}}\)
\( \newcommand{\id}{\mathrm{id}}\)
\( \newcommand{\Span}{\mathrm{span}}\)
\( \newcommand{\kernel}{\mathrm{null}\,}\)
\( \newcommand{\range}{\mathrm{range}\,}\)
\( \newcommand{\RealPart}{\mathrm{Re}}\)
\( \newcommand{\ImaginaryPart}{\mathrm{Im}}\)
\( \newcommand{\Argument}{\mathrm{Arg}}\)
\( \newcommand{\norm}[1]{\| #1 \|}\)
\( \newcommand{\inner}[2]{\langle #1, #2 \rangle}\)
\( \newcommand{\Span}{\mathrm{span}}\) \( \newcommand{\AA}{\unicode[.8,0]{x212B}}\)
\( \newcommand{\vectorA}[1]{\vec{#1}} % arrow\)
\( \newcommand{\vectorAt}[1]{\vec{\text{#1}}} % arrow\)
\( \newcommand{\vectorB}[1]{\overset { \scriptstyle \rightharpoonup} {\mathbf{#1}} } \)
\( \newcommand{\vectorC}[1]{\textbf{#1}} \)
\( \newcommand{\vectorD}[1]{\overrightarrow{#1}} \)
\( \newcommand{\vectorDt}[1]{\overrightarrow{\text{#1}}} \)
\( \newcommand{\vectE}[1]{\overset{-\!-\!\rightharpoonup}{\vphantom{a}\smash{\mathbf {#1}}}} \)
\( \newcommand{\vecs}[1]{\overset { \scriptstyle \rightharpoonup} {\mathbf{#1}} } \)
\( \newcommand{\vecd}[1]{\overset{-\!-\!\rightharpoonup}{\vphantom{a}\smash {#1}}} \)
\(\newcommand{\avec}{\mathbf a}\) \(\newcommand{\bvec}{\mathbf b}\) \(\newcommand{\cvec}{\mathbf c}\) \(\newcommand{\dvec}{\mathbf d}\) \(\newcommand{\dtil}{\widetilde{\mathbf d}}\) \(\newcommand{\evec}{\mathbf e}\) \(\newcommand{\fvec}{\mathbf f}\) \(\newcommand{\nvec}{\mathbf n}\) \(\newcommand{\pvec}{\mathbf p}\) \(\newcommand{\qvec}{\mathbf q}\) \(\newcommand{\svec}{\mathbf s}\) \(\newcommand{\tvec}{\mathbf t}\) \(\newcommand{\uvec}{\mathbf u}\) \(\newcommand{\vvec}{\mathbf v}\) \(\newcommand{\wvec}{\mathbf w}\) \(\newcommand{\xvec}{\mathbf x}\) \(\newcommand{\yvec}{\mathbf y}\) \(\newcommand{\zvec}{\mathbf z}\) \(\newcommand{\rvec}{\mathbf r}\) \(\newcommand{\mvec}{\mathbf m}\) \(\newcommand{\zerovec}{\mathbf 0}\) \(\newcommand{\onevec}{\mathbf 1}\) \(\newcommand{\real}{\mathbb R}\) \(\newcommand{\twovec}[2]{\left[\begin{array}{r}#1 \\ #2 \end{array}\right]}\) \(\newcommand{\ctwovec}[2]{\left[\begin{array}{c}#1 \\ #2 \end{array}\right]}\) \(\newcommand{\threevec}[3]{\left[\begin{array}{r}#1 \\ #2 \\ #3 \end{array}\right]}\) \(\newcommand{\cthreevec}[3]{\left[\begin{array}{c}#1 \\ #2 \\ #3 \end{array}\right]}\) \(\newcommand{\fourvec}[4]{\left[\begin{array}{r}#1 \\ #2 \\ #3 \\ #4 \end{array}\right]}\) \(\newcommand{\cfourvec}[4]{\left[\begin{array}{c}#1 \\ #2 \\ #3 \\ #4 \end{array}\right]}\) \(\newcommand{\fivevec}[5]{\left[\begin{array}{r}#1 \\ #2 \\ #3 \\ #4 \\ #5 \\ \end{array}\right]}\) \(\newcommand{\cfivevec}[5]{\left[\begin{array}{c}#1 \\ #2 \\ #3 \\ #4 \\ #5 \\ \end{array}\right]}\) \(\newcommand{\mattwo}[4]{\left[\begin{array}{rr}#1 \amp #2 \\ #3 \amp #4 \\ \end{array}\right]}\) \(\newcommand{\laspan}[1]{\text{Span}\{#1\}}\) \(\newcommand{\bcal}{\cal B}\) \(\newcommand{\ccal}{\cal C}\) \(\newcommand{\scal}{\cal S}\) \(\newcommand{\wcal}{\cal W}\) \(\newcommand{\ecal}{\cal E}\) \(\newcommand{\coords}[2]{\left\{#1\right\}_{#2}}\) \(\newcommand{\gray}[1]{\color{gray}{#1}}\) \(\newcommand{\lgray}[1]{\color{lightgray}{#1}}\) \(\newcommand{\rank}{\operatorname{rank}}\) \(\newcommand{\row}{\text{Row}}\) \(\newcommand{\col}{\text{Col}}\) \(\renewcommand{\row}{\text{Row}}\) \(\newcommand{\nul}{\text{Nul}}\) \(\newcommand{\var}{\text{Var}}\) \(\newcommand{\corr}{\text{corr}}\) \(\newcommand{\len}[1]{\left|#1\right|}\) \(\newcommand{\bbar}{\overline{\bvec}}\) \(\newcommand{\bhat}{\widehat{\bvec}}\) \(\newcommand{\bperp}{\bvec^\perp}\) \(\newcommand{\xhat}{\widehat{\xvec}}\) \(\newcommand{\vhat}{\widehat{\vvec}}\) \(\newcommand{\uhat}{\widehat{\uvec}}\) \(\newcommand{\what}{\widehat{\wvec}}\) \(\newcommand{\Sighat}{\widehat{\Sigma}}\) \(\newcommand{\lt}{<}\) \(\newcommand{\gt}{>}\) \(\newcommand{\amp}{&}\) \(\definecolor{fillinmathshade}{gray}{0.9}\)Learning Objectives
By the end of this section, you will be able to:
- Describe what the universe was like during the first few minutes after it began to expand
- Explain how the first new elements were formed during the first few minutes after the Big Bang
- Describe how the contents of the universe change as the temperature of the universe decreases
- Apply Wien’s law to analyze radiation emitted by a blackbody
- Explain why we can observe the afterglow of the hot, early universe
- Summarize our current knowledge of the basic properties of the universe including its age and contents
- Describe the chemical building blocks required for life
- Describe the molecular systems and processes driving the origin and evolution of life
- Describe the characteristics of a habitable environment
- Describe some of the extreme conditions on Earth, and explain how certain organisms have adapted to these conditions
The best evidence we have today indicates that the first galaxies did not begin to form until a few hundred million years after the Big Bang. What were things like before there were galaxies and space had not yet stretched very significantly? Amazingly, scientists have been able to calculate in some detail what was happening in the universe in the first few minutes after the Big Bang, which has led us to better understand how the chemicals that shape our environment got to Earth in the first place.
The History of the Idea
It is one thing to say the universe had a beginning (as the equations of general relativity imply) and quite another to describe that beginning. The Belgian priest and cosmologist Georges Lemaître was probably the first to propose a specific model for the Big Bang itself (Figure \(\PageIndex{1}\)). He envisioned all the matter of the universe starting in one great bulk he called the primeval atom, which then broke into tremendous numbers of pieces. Each of these pieces continued to fragment further until they became the present atoms of the universe, created in a vast nuclear fission. In a popular account of his theory, Lemaître wrote, “The evolution of the world could be compared to a display of fireworks just ended—some few red wisps, ashes, and smoke. Standing on a well-cooled cinder, we see the slow fading of the suns and we try to recall the vanished brilliance of the origin of the worlds.”
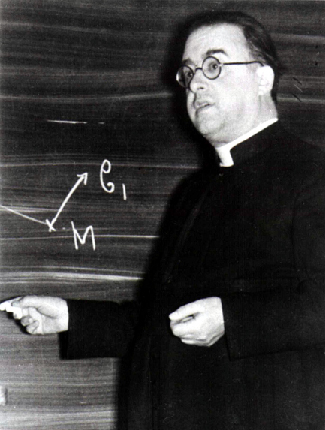
Physicists today know much more about nuclear physics than was known in the 1920s, and they have shown that the primeval fission model cannot be correct. Yet Lemaître’s vision was in some respects quite prophetic. We still believe that everything was together at the beginning; it was just not in the form of matter we now know. Basic physical principles tell us that when the universe was much denser, it was also much hotter, and that it cools as it expands, much as gas cools when sprayed from an aerosol can.
By the 1940s, scientists knew that fusion of hydrogen into helium was the source of the Sun’s energy. Fusion requires high temperatures, and the early universe must have been hot. Based on these ideas, American physicist George Gamow (Figure \(\PageIndex{2}\)) suggested a universe with a different kind of beginning that involved nuclear fusion instead of fission. Ralph Alpher worked out the details for his PhD thesis, and the results were published in 1948. (Gamow, who had a quirky sense of humor, decided at the last minute to add the name of physicist Hans Bethe to their paper, so that the coauthors on this paper about the beginning of things would be Alpher, Bethe, and Gamow, a pun on the first three letters of the Greek alphabet: alpha, beta, and gamma.) Gamow’s universe started with fundamental particles that built up the heavy elements by fusion in the Big Bang.
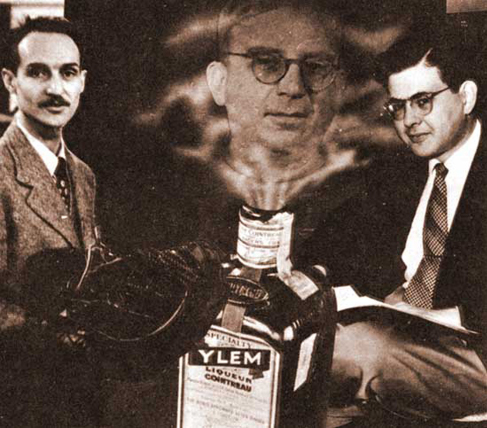
Gamow’s ideas were close to our modern view, except we now know that the early universe remained hot enough for fusion for only a short while. Thus, only the three lightest elements—hydrogen, helium, and a small amount of lithium—were formed in appreciable abundances at the beginning. The heavier elements formed later in stars. Since the 1940s, many astronomers and physicists have worked on a detailed theory of what happened in the early stages of the universe.
The second step in understanding the evolution of the universe is to realize that at very early times, it was so hot that it contained mostly radiation (and not the matter that we see today). The photons that filled the universe could collide and produce material particles; that is, under the conditions just after the Big Bang, energy could turn into matter (and matter could turn into energy). We can calculate how much mass is produced from a given amount of energy by using Einstein’s formula \(E = mc^2\).
The idea that energy could turn into matter in the universe at large is a new one for many students, since it is not part of our everyday experience. That’s because, when we compare the universe today to what it was like right after the Big Bang, we live in cold, hard times. The photons in the universe today typically have far-less energy than the amount required to make new matter. This process has been observed in particle accelerators around the world. If we have enough energy, under the right circumstances, new particles of matter (and antimatter) are indeed created —and the conditions were right during the first few minutes after the expansion of the universe began.
The Evolution of the Early Universe
Keeping these three ideas in mind, we can trace the evolution of the universe from the time it was about 0.01 second old and had a temperature of about 100 billion K. Why not begin at the very beginning? There are as yet no theories that allow us penetrate to a time before about \(10^{–43}\) second (this number is a decimal point followed by 42 zeros and then a one). It is so small that we cannot relate it to anything in our everyday experience. When the universe was that young, its density was so high that the theory of general relativity is not adequate to describe it, and even the concept of time breaks down.
Scientists, by the way, have been somewhat more successful in describing the universe when it was older than \(10^{–43}\) second but still less than about 0.01 second old. We will take a look at some of these ideas later in this chapter, but for now, we want to start with somewhat more familiar situations.
By the time the universe was 0.01 second old, it consisted of a soup of matter and radiation; the matter included protons and neutrons, leftovers from an even younger and hotter universe. Each particle collided rapidly with other particles. The temperature was no longer high enough to allow colliding photons to produce neutrons or protons, but it was sufficient for the production of electrons and positrons (Figure \(\PageIndex{3}\)). There was probably also a sea of exotic subatomic particles that would later play a role as dark matter. All the particles jiggled about on their own; it was still much too hot for protons and neutrons to combine to form the nuclei of atoms.
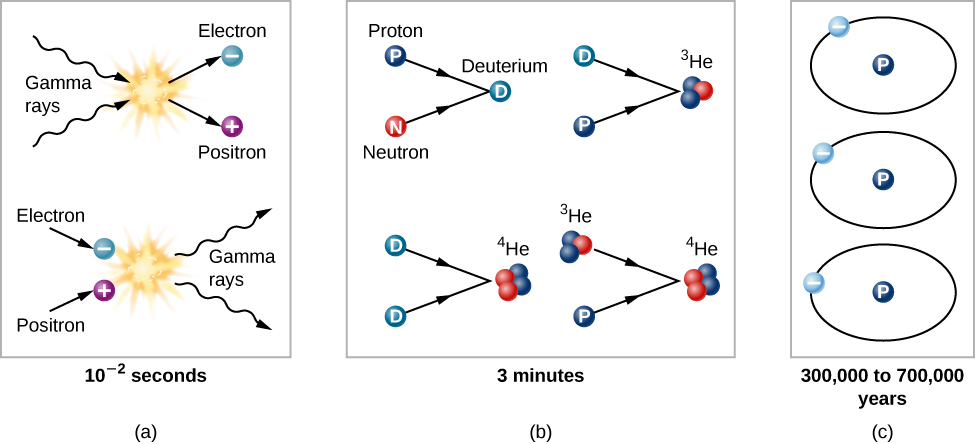
Think of the universe at this time as a seething cauldron, with photons colliding and interchanging energy, and sometimes being destroyed to create a pair of particles. The particles also collided with one another. Frequently, a matter particle and an antimatter particle met and turned each other into a burst of gamma-ray radiation.
By the time the universe was a little more than 1 second old, the density had dropped to the point where neutrinos no longer interacted with matter but simply traveled freely through space. In fact, these neutrinos should now be all around us. Since they have been traveling through space unimpeded (and hence unchanged) since the universe was 1 second old, measurements of their properties would offer one of the best tests of the Big Bang model. Unfortunately, the very characteristic that makes them so useful—the fact that they interact so weakly with matter that they have survived unaltered for all but the first second of time—also renders them unable to be measured, at least with present techniques. Perhaps someday someone will devise a way to capture these elusive messengers from the past.
Atomic Nuclei Form
When the universe was about 3 minutes old and its temperature was down to about 900 million K, protons and neutrons could combine. At higher temperatures, these atomic nuclei had immediately been blasted apart by interactions with high-energy photons and thus could not survive. But at the temperatures and densities reached between 3 and 4 minutes after the beginning, deuterium (a proton and neutron) lasted long enough that collisions could convert some of it into helium, (Figure \(\PageIndex{4}\)). In essence, the entire universe was acting the way centers of stars do today—fusing new elements from simpler components. In addition, a little bit of element 3, lithium, could also form.
This burst of cosmic fusion was only a brief interlude, however. By 4 minutes after the Big Bang, more helium was having trouble forming. The universe was still expanding and cooling down. After the formation of helium and some lithium, the temperature had dropped so low that the fusion of helium nuclei into still-heavier elements could not occur. No elements beyond lithium could form in the first few minutes. That 4-minute period was the end of the time when the entire universe was a fusion factory. In the cool universe we know today, the fusion of new elements is limited to the centers of stars and the explosions of supernovae.
Still, the fact that the Big Bang model allows the creation of a good deal of helium is the answer to a long-standing mystery in astronomy. Put simply, there is just too much helium in the universe to be explained by what happens inside stars. All the generations of stars that have produced helium since the Big Bang cannot account for the quantity of helium we observe. Furthermore, even the oldest stars and the most distant galaxies show significant amounts of helium. These observations find a natural explanation in the synthesis of helium by the Big Bang itself during the first few minutes of time. We estimate that 10 times more helium was manufactured in the first 4 minutes of the universe than in all the generations of stars during the succeeding 10 to 15 billion years.
These nice animations that explain the way in which different elements formed in the history of the universe are from the University of Chicago’s Origins of the Elements site.
Learning from Deuterium
We can learn many things from the way the early universe made atomic nuclei. It turns out that all of the deuterium (a hydrogen nucleus with a neutron in it) in the universe was formed during the first 4 minutes. In stars, any region hot enough to fuse two protons to form a deuterium nucleus is also hot enough to change it further—either by destroying it through a collision with an energetic photon or by converting it into helium through nuclear reactions.
The amount of deuterium that can be produced in the first 4 minutes of creation depends on the density of the universe at the time deuterium was formed. If the density were relatively high, nearly all the deuterium would have been converted into helium through interactions with protons, just as it is in stars. If the density were relatively low, then the universe would have expanded and thinned out rapidly enough that some deuterium would have survived. The amount of deuterium we see today thus gives us a clue to the density of the universe when it was about 4 minutes old. Theoretical models can relate the density then to the density now; thus, measurements of the abundance of deuterium today can give us an estimate of the current density of the universe.
The measurements of deuterium indicate that the present-day density of ordinary matter—protons and neutrons—is about \(5 \times 10^{–28} \text{ kg/m}^3\). Deuterium can only provide an estimate of the density of ordinary matter because the abundance of deuterium is determined by the particles that interact to form it, namely protons and neutrons alone. From the abundance of deuterium, we know that not enough protons and neutrons are present, by a factor of about 20, to produce a critical-density universe.
We do know, however, that there are dark matter particles that add to the overall matter density of the universe, which is then higher than what is calculated for ordinary matter alone. Because dark matter particles do not affect the production of deuterium, measurement of the deuterium abundance cannot tell us how much dark matter exists. Dark matter is made of some exotic kind of particle, not yet detected in any earthbound laboratory. It is definitely not made of protons and neutrons like the readers of this book.
The description of the first few minutes of the universe is based on theoretical calculations. It is crucial, however, that a scientific theory should be testable. What predictions does it make? And do observations show those predictions to be accurate? One success of the theory of the first few minutes of the universe is the correct prediction of the amount of helium in the universe.
Another prediction is that a significant milestone in the history of the universe occurred about 380,000 years after the Big Bang. Scientists have directly observed what the universe was like at this early stage, and these observations offer some of the strongest support for the Big Bang theory. To find out what this milestone was, let’s look at what theory tells us about what happened during the first few hundred thousand years after the Big Bang.
The fusion of helium and lithium was completed when the universe was about 4 minutes old. The universe then continued to resemble the interior of a star in some ways for a few hundred thousand years more. It remained hot and opaque, with radiation being scattered from one particle to another. It was still too hot for electrons to “settle down” and become associated with a particular nucleus; such free electrons are especially effective at scattering photons, thus ensuring that no radiation ever got very far in the early universe without having its path changed. In a way, the universe was like an enormous crowd right after a popular concert; if you get separated from a friend, even if he is wearing a flashing button, it is impossible to see through the dense crowd to spot him. Only after the crowd clears is there a path for the light from his button to reach you.
The Universe Becomes Transparent
Not until a few hundred thousand years after the Big Bang, when the temperature had dropped to about 3000 K and the density of atomic nuclei to about 1000 per cubic centimeter, did the electrons and nuclei manage to combine to form stable atoms of hydrogen and helium Figure \(\PageIndex{3}\). With no free electrons to scatter photons, the universe became transparent for the first time in cosmic history. From this point on, matter and radiation interacted much less frequently; we say that they decoupled from each other and evolved separately. Suddenly, electromagnetic radiation could really travel, and it has been traveling through the universe ever since. But before we get too deep into this, let's review Blackbody emitters.
All bodies emit electromagnetic radiation over a range of wavelengths. In an earlier chapter, we learned that a cooler body radiates less energy than a warmer body. We also know by observation that when a body is heated and its temperature rises, the perceived wavelength of its emitted radiation changes from infrared to red, and then from red to orange, and so forth. As its temperature rises, the body glows with the colors corresponding to ever-smaller wavelengths of the electromagnetic spectrum. This is the underlying principle of the incandescent light bulb: A hot metal filament glows red, and when heating continues, its glow eventually covers the entire visible portion of the electromagnetic spectrum. The temperature (T) of the object that emits radiation, or the emitter, determines the wavelength at which the radiated energy is at its maximum. For example, the Sun, whose surface temperature is in the range between 5000 K and 6000 K, radiates most strongly in a range of wavelengths about 560 nm in the visible part of the electromagnetic spectrum. Your body, when at its normal temperature of about 300 K, radiates most strongly in the infrared part of the spectrum.
Radiation that is incident on an object is partially absorbed and partially reflected. At thermodynamic equilibrium, the rate at which an object absorbs radiation is the same as the rate at which it emits it. Therefore, a good absorber of radiation (any object that absorbs radiation) is also a good emitter. A perfect absorber absorbs all electromagnetic radiation incident on it; such an object is called a blackbody.

Although the blackbody is an idealization, because no physical object absorbs 100% of incident radiation, we can construct a close realization of a blackbody in the form of a small hole in the wall of a sealed enclosure known as a cavity radiator, as shown in Figure \(\PageIndex{4}\). The inside walls of a cavity radiator are rough and blackened so that any radiation that enters through a tiny hole in the cavity wall becomes trapped inside the cavity. At thermodynamic equilibrium (at temperature T), the cavity walls absorb exactly as much radiation as they emit. Furthermore, inside the cavity, the radiation entering the hole is balanced by the radiation leaving it. The emission spectrum of a blackbody can be obtained by analyzing the light radiating from the hole. Electromagnetic waves emitted by a blackbody are called blackbody radiation.
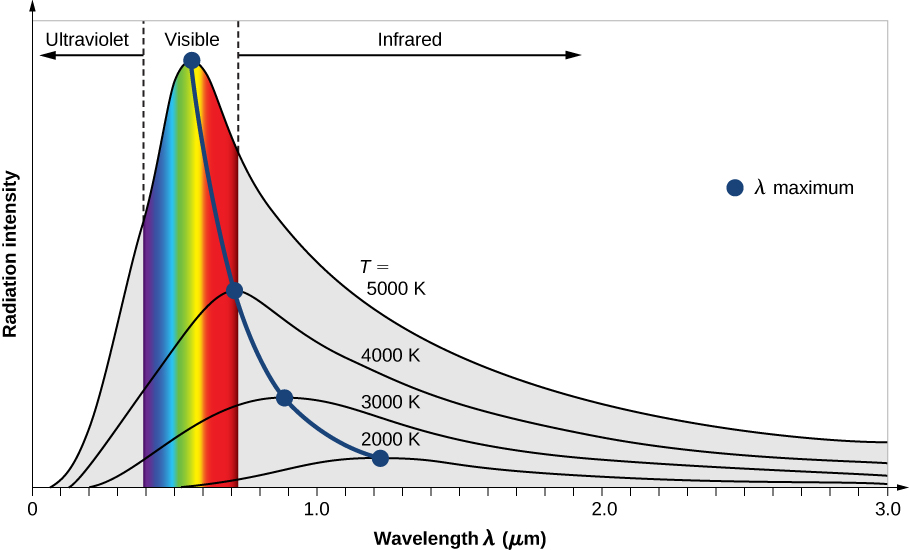
The intensity \(I(\lambda, T)\) of blackbody radiation depends on the wavelength \(\lambda\) of the emitted radiation and on the temperature T of the blackbody (Figure \(\PageIndex{5}\)). The function \(I(\lambda, T)\) is the power intensity that is radiated per unit wavelength; in other words, it is the power radiated per unit area of the hole in a cavity radiator per unit wavelength. According to this definition, \(I(\lambda, T)d\lambda\) is the power per unit area that is emitted in the wavelength interval from \(\lambda\) to \(\lambda + d\lambda\). The intensity distribution among wavelengths of radiation emitted by cavities was studied experimentally at the end of the nineteenth century. Generally, radiation emitted by materials only approximately follows the blackbody radiation curve (Figure \(\PageIndex{6}\)); however, spectra of common stars do follow the blackbody radiation curve very closely.
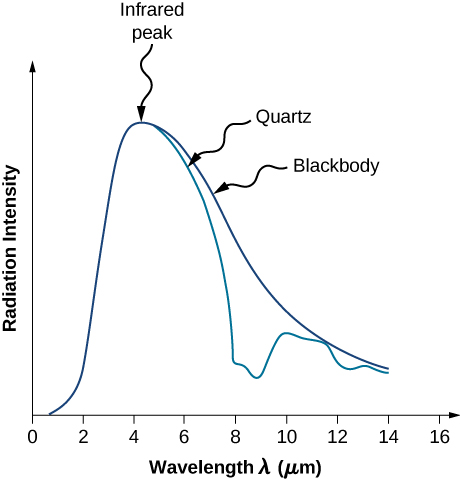
Two important laws summarize the experimental findings of blackbody radiation: Wien’s displacement law and Stefan’s law (which is beyond the scope of our course). Wien’s displacement law is illustrated in Figure \(\PageIndex{5}\) by the curve connecting the maxima on the intensity curves. In these curves, we see that the hotter the body, the shorter the wavelength corresponding to the emission peak in the radiation curve. Quantitatively, Wien’s law reads
\[\lambda_{max}T = 2.898 \times 10^{-3} m \cdot K \label{Wien}\]
where \(\lambda_{max}\) is the position of the maximum in the radiation curve. In other words, \(\lambda_{max}\) is the wavelength at which a blackbody radiates most strongly at a given temperature T. Note that in Equation \ref{Wien}, the temperature is in kelvins. Wien’s displacement law allows us to estimate the temperatures of distant stars by measuring the wavelength of radiation they emit.
Example \(\PageIndex{1}\): Temperatures of Distant Stars
On a clear evening during the winter months, if you happen to be in the Northern Hemisphere and look up at the sky, you can see the constellation Orion (The Hunter). One star in this constellation, Rigel, flickers in a blue color and another star, Betelgeuse, has a reddish color, as shown in Figure \(\PageIndex{4}\). Which of these two stars is cooler, Betelgeuse or Rigel?
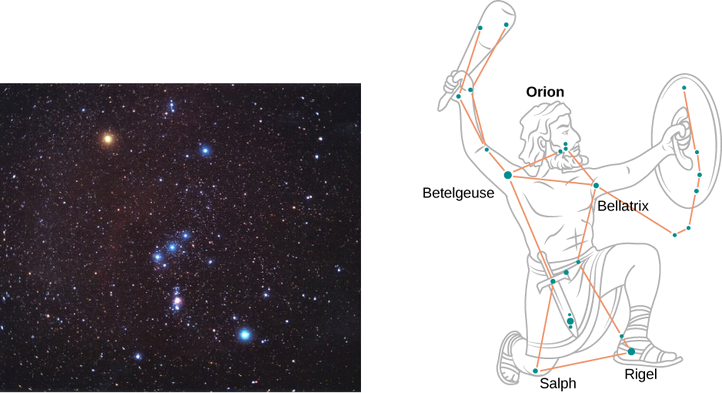
Strategy
We treat each star as a blackbody. Then according to Wien’s law, its temperature is inversely proportional to the wavelength of its peak intensity. The wavelength \(\lambda_{max}^{(blue)}\) of blue light is shorter than the wavelength \(\lambda_{max}^{(red)}\) of red light. Even if we do not know the precise wavelengths, we can still set up a proportion.
Solution
Writing Wien’s law for the blue star and for the red star, we have
\[\begin {align*} \lambda_{max}^{(red)}T_{(red)} &= 2.898 \times 10^{-3} m \cdot K \\[5pt] &= \lambda_{max}^{(blue)} T_{(blue)} \end{align*}\]
When simplified, this gives
\[T_{(red)} = \dfrac{\lambda_{max}^{(blue)}}{\lambda_{max}^{(red)}}T_{(blue)} < T_{(blue)} \nonumber \]
Therefore, Betelgeuse is cooler than Rigel.
Significance
Note that Wien’s displacement law tells us that the higher the temperature of an emitting body, the shorter the wavelength of the radiation it emits. The qualitative analysis presented in this example is generally valid for any emitting body, whether it is a big object such as a star or a small object such as the glowing filament in an incandescent lightbulb.
Exercise \(\PageIndex{1}\)
The flame of a peach-scented candle has a yellowish color and the flame of a Bunsen’s burner in a chemistry lab has a bluish color. Which flame has a higher temperature?
- Answer
Discovery of the Cosmic Background Radiation
If the model of the universe described here is correct, then—as we look far outward in the universe and thus far back in time—the first “afterglow” of the hot, early universe should still be detectable. Observations of it would be very strong evidence that our theoretical calculations about how the universe evolved are correct. As we shall see, we have indeed detected the radiation emitted at this photon decoupling time, when radiation began to stream freely through the universe without interacting with matter (Figure \(\PageIndex{7}\)).
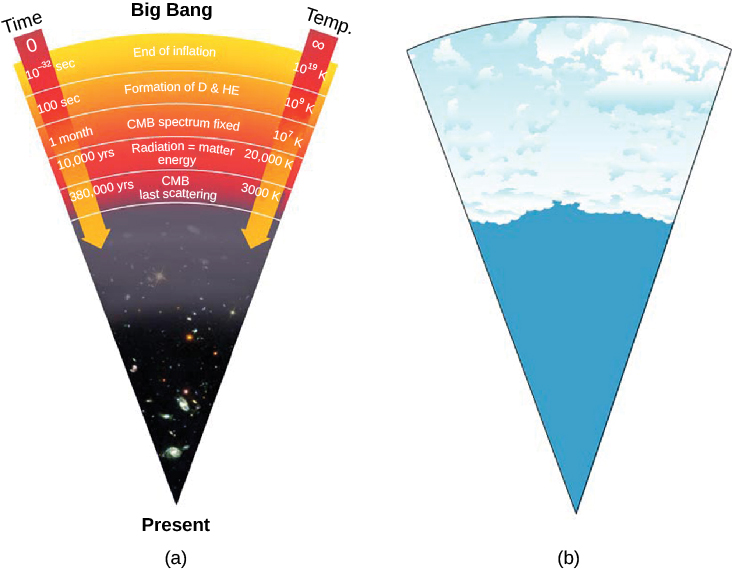
The detection of this afterglow was initially an accident. In the late 1940s, Ralph Alpher and Robert Herman, working with George Gamow, realized that just before the universe became transparent, it must have been radiating like a blackbody at a temperature of about 3000 K—the temperature at which hydrogen atoms could begin to form. If we could have seen that radiation just after neutral atoms formed, it would have resembled radiation from a reddish star. It was as if a giant fireball filled the whole universe.
But that was nearly 14 billion years ago, and, in the meantime, the scale of the universe has increased a thousand fold. This expansion has increased the wavelength of the radiation by a factor of 1000. According to Wien’s law, which relates wavelength and temperature, the expansion has correspondingly lowered the temperature by a factor of 1000.
Alpher and Herman predicted that the glow from the fireball should now be at radio wavelengths and should resemble the radiation from a blackbody at a temperature only a few degrees above absolute zero. Since the fireball was everywhere throughout the universe, the radiation left over from it should also be everywhere. If our eyes were sensitive to radio wavelengths, the whole sky would appear to glow very faintly. However, our eyes can’t see at these wavelengths, and at the time Alpher and Herman made their prediction, there were no instruments that could detect the glow. Over the years, their prediction was forgotten.
In the mid-1960s, in Holmdel, New Jersey, Arno Penzias and Robert Wilson of AT&T’s Bell Laboratories had built a delicate microwave antenna (Figure \(\PageIndex{8}\)) to measure astronomical sources, including supernova remnants. They were plagued with some unexpected background noise, just like faint static on a radio, which they could not get rid of. The puzzling thing about this radiation was that it seemed to be coming from all directions at once, which was unusual. Nothing the scientists did could reduce the background radiation to zero, and they reluctantly came to accept that it must be real, and it must be coming from space.
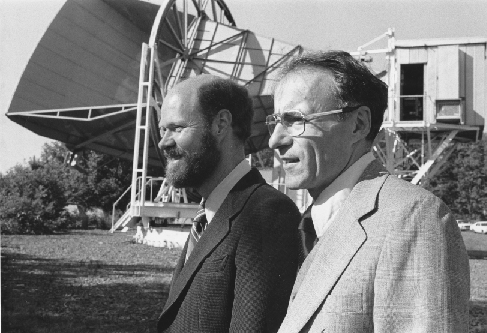
Penzias and Wilson were not cosmologists, but as they began to discuss their puzzling discovery with other scientists, they were quickly put in touch with a group of astronomers and physicists at Princeton University (a short drive away). These astronomers had—as it happened—been redoing the calculations of Alpher and Herman from the 1940s and also realized that the radiation from the decoupling time should be detectable as a faint afterglow of radio waves. The different calculations of what the observed temperature would be for this cosmic microwave background (CMB)1 were uncertain, but all predicted less than 40 K.
Penzias and Wilson found the distribution of intensity at different radio wavelengths to correspond to a temperature of 3.5 K. This is very cold—closer to absolute zero than most other astronomical measurements—and a testament to how much space (and the waves within it) has stretched. Their measurements have been repeated with better instruments, which give us a reading of 2.73 K. So Penzias and Wilson came very close. Rounding this value, scientists often refer to “the 3-degree microwave background.”
Many other experiments on Earth and in space soon confirmed the discovery by Penzias and Wilson: The radiation was indeed coming from all directions (it was isotropic) and matched the predictions of the Big Bang theory with remarkable precision. Penzias and Wilson had inadvertently observed the glow from the primeval fireball. They received the Nobel Prize for their work in 1978. And just before his death in 1966, Lemaître learned that his “vanished brilliance” had been discovered and confirmed.
Properties of the Cosmic Microwave Background
One issue that worried astronomers is that Penzias and Wilson were measuring the background radiation filling space through Earth’s atmosphere. What if that atmosphere is a source of radio waves or somehow affected their measurements? It would be better to measure something this important from space.
The first accurate measurements of the CMB were made with a satellite orbiting Earth. Named the Cosmic Background Explorer (COBE), it was launched by NASA in November 1989. The data it received quickly showed that the CMB closely matches that expected from a blackbody with a temperature of 2.73 K (Figure \(\PageIndex{9}\)). This is exactly the result expected if the CMB was indeed redshifted radiation emitted by a hot gas that filled all of space shortly after the universe began
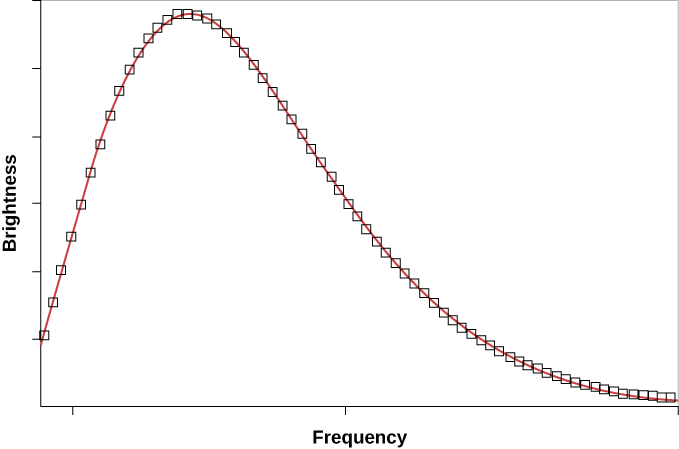
The first important conclusion from measurements of the CMB, therefore, is that the universe we have today has indeed evolved from a hot, uniform state. This observation also provides direct support for the general idea that we live in an evolving universe, since the universe is cooler today than it was in the beginning.
After the CMB was emitted, the universe continued to expand and cool off. By 400 to 500 million years after the Big Bang, the very first stars and galaxies had already formed. Deep in the interiors of stars, matter was reheated, nuclear reactions were ignited, and the more gradual synthesis of the heavier elements that we have discussed throughout this book began.
We conclude this quick tour of our model of the early universe with a reminder. You must not think of the Big Bang as a localized explosion in space, like an exploding superstar. There were no boundaries and there was no single site where the explosion happened. It was an explosion of space (and time and matter and energy) that happened everywhere in the universe. All matter and energy that exist today, including the particles of which you are made, came from the Big Bang. We were, and still are, in the midst of a Big Bang; it is all around us.
The Chemical Origins of Earth
The earth has been in a state of continual change since its formation. The major part of this change, involving volcanism and tectonics, has been driven by heat produced from the decay of radioactive elements within the earth. The other source of change has been solar energy, which acts as the driving force of weathering and is the ultimate source of energy for living organisms.
The solar system was probably formed about 4.6 billion years ago, and the oldest known rocks have an age of 3.8 billion years. There is thus a gap of 0.8 billion years for which there is no direct evidence. It is known that the earth was subjected to extensive bombardment earlier in its history; recent computer simulations suggest that the moon could have resulted from an especially massive collision with another body. Although these major collisions have diminished in magnitude as the matter in the solar system has become more consolidated, they continue to occur, with the most recent one being responsible for the annihilation of the dinosaurs and much of the other life on Earth. The lack of many overt signs of these collisions (such as craters, for example) testifies to the dynamic processes at work on the Earth’s surface and beneath it.
Chemical Composition of the Earth
The earth is composed of 90 chemical elements, of which 81 have at least one stable isotope. The unstable elements are 43Tc and 61Pm, and all elements heavier than 83Bi.
Note that the vertical axis is logarithmic, which has the effect of greatly reducing the visual impression of the differences between the various elements.
The chart gives the abundances of the elements present in the solar system, in the earth as a whole, and in the various geospheres. Of particular interest are the differences between the terrestrial and cosmic abundances, which are especially notable in the cases of the lighter elements (H, C, N) and the noble gas elements (He, Ne, Ar, Xe, Kr).
Example \(\PageIndex{2}\)
Given the mix of elements that are present in the earth, how might they combine so as to produce the chemical composition we now observe?
Solution
Given the mix of elements that are present in the earth, how might they combine so as to produce the chemical composition we now observe? Thermodynamics allows us to predict the composition that any isolated system will eventually reach at a given temperature and pressure. Of course the earth is not an isolated system, although most parts of it can be considered approximately so in many respects, on time scales sufficient to make thermodynamic predictions reasonably meaningful. The equilibrium states predicted by thermodynamics differ markedly from the observed compositions. The atmosphere, for example, contains 0.03% \(\ce{CO2}\), 78% \(\ce{N2}\) and 21% \(\ce{O2}\); in a world at equilibrium the air would be 99% \(\ce{CO2}\).
Similarly, the oceans, containing about 3.5% NaCl, would have a salt content of 35% if they were in equilibrium with the atmosphere and the lithosphere. Trying to understand the mechanisms that maintain these non-equilibrium states is an important part of contemporary environmental geochemistry.
Structure of the Earth
Studies based on the reflection and refraction of the acoustic waves resulting from earthquakes show that the interior of the earth consists of four distinct regions. A combination of physical and chemical processes led to the differentiation of the earth into these major parts. This is believed to have occurred approximately 4 billion years ago.
The Earth's Core
The Earth’s core is believed to consist of two regions. The inner core is solid, while the outer core is liquid. This phase difference probably reflects a difference in pressure and composition, rather than one of temperature. Density estimates obtained from seismological studies indicate that the core is metallic, and mainly iron, with 8-10 percent of lighter elements.
Hypotheses about the nature of the core must be consistent with the the core’s role as the source of the earth’s magnetic field. This field arises from convective motion of the electrically conductive liquid comprising the outer core. Whether this convection is driven by differences in temperature or composition is not certain. The estimated abundance of radioactive isotopes (mainly U238 and K40 in the core is sufficient to provide the thermal energy required to drive the convective dynamo. Laboratory experiments on the high-pressure behavior of iron oxides and sulfides indicate that these substances are probably metallic in nature, and hence conductive, at the temperatures (4000-5000K) and pressures (1.3-3.5 million atm) that are estimated for the core. Their presence in the core, alloyed with the iron, would be consistent with the observed density, and would also resolve the apparent lack of sulfur in the earth, compared to its primordial abundance.
The mantle
The region extending from the outer part of the core to the crust of the earth is known as the mantle. The mantle is composed of oxides and silicates, i.e., of rock. It was once believed that this rock was molten, and served as a source of volcanic magma. It is now known on the basis of seismological evidence that the mantle is not in the liquid state. Laboratory experiments have shown, however, that when rock is subjected to the high temperatures and pressures believed to exist in the mantle, it can be deformed and flows very much like a liquid.
The upper part of the mantle consists of a region of convective cells whose motion is driven by the heat due to decay of radioactive potassium, thorium, and uranium, which were selectively incorporated in the crystal lattices of the lower-density minerals that form the mantle. There are several independent sources of evidence of this motion. First, there are gravitational anomalies; the force of gravity, measured by changes in elevation in the sea surface, is different over upward and downward moving regions, and has permitted the mapping of some of the convective cells. Secondly, numerous isotopic ratio studies have traced the exchange of material between oceanic sediments, upper mantle rock, and back into the continental crust, which forms from melting of the upper mantle. Thirdly, the composition of the basalt formed by upper mantle melting is quite uniform everywhere, suggesting complete mixing of diverse materials incorporated into the mantle over periods of 100 million years.
High-pressure studies in the laboratory have revealed that olivine, a highly abundant substance in the mantle composed of Fe, Mg, Si, and O (and also the principal constituent of meteorites) can undergo a reversible phase change between two forms differing in density. Estimates of conditions within the upper mantle suggest that the this phase change could occur within this region in such as way as to contribute to convection. The most apparent effect of mantle convection is the motion it imparts to the earth’s crust, as evidenced by the the external topography of the earth.
The crust
The outermost part of the earth, known also as the lithosphere, is broken up into plates that are supported by the underlying mantle, and are moved by the convective cells within the mantle at a rate of a few centimetres per year. New crust is formed where plates move away from each other under the oceans, and old crust is recycled back into the mantle as where plates moving in opposite directions collide.
The oceanic crust
The parts of the crust that contain the world’s oceans are very different from the parts that form the continents. The continental crust is 10-70 km thick, while oceanic crust averages only 5-7 km in thickness. Oceanic crust is more dense (3.0-3.1 g cm–3) and therefore “floats” on the mantle at a greater depth than does continental crust (density 2.7-2.8 ). Finally, oceanic crust is much younger; the oldest oceanic crust is about 200 million years old, while the most ancient continental rocks were formed 3.8 billion years ago.
New crust is formed from molten material in the upper mantle at the divergent boundaries that exist at undersea ridges. The melting is due to the rise in temperature associated with the nearly adiabatic decompression of the upper 50-70 km of mantle material as separation of the plates reduces the pressure below. The molten material collects in a magma pocket which is gradually exuded in undersea lava flows. The solidified lava is transformed into crust by the effects of heat and the action of seawater which selectively dissolves the more soluble components. | |||||
![]() |
|||||
An animated view of seafloor spreading. |
|||||
Plate collisions
Where two plates collide, one generally plunges under the other and returns to the mantle in a process known as subduction. Since the continental plates have a lower density, they tend to float above the oceanic plates and resist subduction. At continental boundaries such as that of the North American west coast where an oceanic plate pushes under the continental crust, oceanic sediments may be sheared off, resulting in a low coastal mountain range (see here for a nice animation of this process.) Also, the injection of water into the subducting material lowers its melting point, resulting in the formation of shallow magma pockets and volcanic activity. Divergent plate boundaries can cross continents, however; temporary divergences create rift valleys such as the Rhine and Rio Grande, while permanent ones eventually lead to new oceanic basins.
Collision of two continental plates can also occur; the most notable example is the one resulting in the formation of the Himalayan mountain chain.
The Earth is composed of 90 chemical elements, of which 81 have at least one stable isotope. Most of these elements have also been detected in stars. Where did these elements come from? The accepted scenario is that the first major element to condense out of the primordial soup was helium , which still comprises about one-quarter of the mass of the known universe.
Hydrogen is the least thermodynamically stable of the elements, and at very high temperatures will combine with itself in a reaction known as nuclear fusion to form the next element, 2He4. "Heavier" nuclei (that is , those having high atomic numbers, indicated here by the subscript preceding the element symbol), are more stable than "lighter" ones, so this fusion process can continue up to 56Fe, which is the most energetically stable of all the nuclides. Beyond this point, heavier nuclei slowly become less stable, so fission becomes more likely. FIssion, however, is not considered an important mechanism of primordialnucleosynthesis, so other processes are invoked, as discussed farther below.
Primordial Chemistry
According to the “big bang” theory for which there is now overwhelming evidence, the universe as we know it (that is, all space, time, and matter) had its origin in a point source or singularity that began an explosive expansion about 12-15 billion years ago, and which is still continuing.
Following a brief period of extremely rapid expansion called inflation, protons and neutrons condensed out of the initial quantum soup after about 10–32 s. Helium and hydrogen became stable during the first few minutes, along with some of the very lightest nuclides up to 7Li, which were formed through various fusion and neutron-absorption processes. Formation of most heavier elements was delayed for about 106 years until nucleosynthesis commenced in the first stars. Hydrogen still accounts for about 93% of the atoms in the universe.
The main lines of observational evidence that support this theory are the 2.7K background radiation that permeates the cosmos (the cooled-down remnants of the initial explosion), and the abundances of the lightest elements. Conventional physics is able to extrapolate back to about the first 10–33 second; what happened before then remains speculative.
Stellar nucleosynthesis
All elements beyond hydrogen were formed in regions where the concentration of matter was large, and the temperature was high; in other words, in stars. The formation of a star begins when the gravitational forces due to a large local concentration of hydrogen bring about a contraction and compression to densities of around 105 g cm–3. This is a highly exothermic process in which the gravitational potential energy is released as heat, about 1200 kJ per gram, raising the temperature to about 107 K. Under these conditions, hydrogen nuclei possess sufficient kinetic energy to overcome their electrostatic repulsion and undergo nuclear fusion:
4 1H1-> 2He4 + 2 b+ + 2 g + 2 n
Hydrogen burning
There will be a net mass loss in above process, which will therefore be highly exothermic and is known as “hydrogen burning”. As hydrogen burning proceeds, the helium collects in the core of the star, raising the density to 108 g cm–3 and the temperature to 108 K. This temperature is high enough to initiate helium burning, which proceeds in several steps:
2 2He4 -> 4Be8 + g
The first product, 4Be8 has a half life of only 10–16 sec, but a sufficient amount accumulates to drive the following two reactions:
4Be8 + 2He4 -> 6C12 + g
6C12 + 1H1 -> 7N13 -> 6C13 + b+ + g
The size of a star depends on the balance between the kinetic energy of its matter and the gravitational attraction of its mass. As the helium burning runs its course, the temperature drops and the star begins to contract. The course of further nucleosynthesis and the subsequent fate of the star itself depends on the star’s mass.
Small stars
If the mass of the star is no greater than 1.4 times the mass of our sun, the star collapses to a white dwarf, and eventually cools to a dark, dense dead star.
Big stars
In larger stars, the gravitational forces are sufficiently strong to overcome the normal repulsion between atoms, and so gravitational collapse continues. The gravitational energy released in this process produces temperatures of 6 108 K, which are sufficient to initiate a complex series of nuclear reactions known as the carbon-nitrogen cycle. The net reaction of this cycle is the further fusion of hydrogen to helium, in which C12 acts as a catalyst, and various nuclides of nitrogen and oxygen are intermediates. The temperature is sufficiently high, however, to initiate fusion reactions of some of these intermediates:
6C12 + 6C12 -> 10Ne20 + 2He4
2 8O16 -> 14Si28 + 2He4
2 8O16 -> 16S31 + 0n1
Supernovas
The intense gamma radiation that is produced in some of these reactions breaks some of the product nuclei into smaller fragments, which can then fuse into a variety of heavier species, up to the limit of26Fe56, beyond which fusion is no longer exothermic. The greater relative abundance of elements such as 6C12, 8O16, and 10Ne20 which differ by a 2He4 nucleus, reflects the participation of the latter species in these processes. These exothermic reactions eventually produce temperatures of 8 109 K, while contraction continues until the central core is essentially a ball of neutrons having a radius of about 10 km and a density of 1014 g cm–3. At the same time the outer shell of the star is blasted away in an explosion known as a supernova.
Since 26Fe56 has the highest binding energy per nucleon of any nuclide, there are no exothermic processes which can lead to the formation of heavier elements. Fusion into heavier species is also precluded by the electrostatic repulsion of the highly charged nuclei. However, the process of neutron capture can still take place (this is the same process that is used to make synthetic elements). The neutrons are by-products of a large variety of stellar processes, and are present in a wide range of energies. Two general types of neutron capture processes are recognized. In an “s” (slow) process, only a single neutron is absorbed and the product usually decomposes by b-decay into a more proton-rich species.
Elements heavier than iron
26Fe56 + 0n1 -> 26Fe56 -> 26Fe59 -> 27Co59 + –1e0
This process occurs at rates of about 105 yr–1 , and accounts for the lighter isotopes of many elements. The other process (the “r”, or rapid process) occurs in regions of high neutron density and involves multiple captures at rates of 0.1-10 sec–1:
26Fe56 + 13 0n1 -> 26Fe56 -> 27Co59 + –1e0
This mechanism favors the heavier, neutron-rich isotopes and the heaviest elements.
Other elements
A few nuclei are not accounted for by any of the processes mentioned. These are all low-abundance species, and they probably result from processes having low rates. Examples are Sn112 and Sn114which may be produced through proton-capture, and H2, Li6, Li7, Be, B10 and B11, which may come from spallation processes resulting from collisions of cosmic ray particles with heavier elements
Formation of the solar system
The solar system is believed to have formed about 5 billion years ago as a result of aggregation of cosmic dust and interstellar atoms in a region of space in which the density of such material happened to be greater than average. Over 99.8% of this mass, which consisted mostly of hydrogen, collapsed into a proto-sun; the gravitational energy released in this process raised the temperature sufficiently to initiate the hydrogen fusion reactions discussed above.
The planets
The remaining material probably formed a disk that rotated around the sun. As the temperature dropped to around 2000K, some of the most stable combinations of the elements began to condense out. These substances might have been calcium aluminum silicates, followed by the more volatile iron-nickel system, and then magnesium silicates. The further aggregation of these materials, together with the other constituents of the cooling disk, is now believed to be the origin of the planets. Density estimates indicate that the planets closest to the sun are predominantly rocky in nature, and probably condensed first. The outer planets (Uranus, Neptune and Pluto) appear to consist largely of water ice, methane, and ammonia, with a smaller rocky core.
Summary
Lemaître, Alpher, and Gamow first worked out the ideas that are today called the Big Bang theory. The universe cools as it expands. The energy of photons is determined by their temperature, and calculations show that in the hot, early universe, photons had so much energy that when they collided with one another, they could produce material particles. As the universe expanded and cooled, protons and neutrons formed first, then came electrons and positrons. Next, fusion reactions produced deuterium, helium, and lithium nuclei. Measurements of the deuterium abundance in today’s universe show that the total amount of ordinary matter in the universe is only about 5% of the critical density.
When the universe became cool enough to form neutral hydrogen atoms, the universe became transparent to radiation. Scientists have detected the cosmic microwave background (CMB) radiation from this time during the hot, early universe. CMB measurements also indicate that the universe is 13.8 billion years old.
The study of life in the universe, including its origin on Earth, is called astrobiology. Life as we know it requires water, certain elemental raw materials (carbon, hydrogen, nitrogen, oxygen, phosphorus, and sulfur), energy, and an environment in which the complex chemistry of life is stable. Carbon-based (or organic) molecules are abundant in space and may also have been produced by processes on Earth. Life appears to have spread around our planet within 400 million years after the end of heavy bombardment, if not sooner. The actual origin of life—the processes leading from chemistry to biology—is not completely understood. Once life took hold, it evolved to use many energy sources, including first a range of different chemistries and later light, and diversified across a range of environmental conditions that humans consider “extreme.” This proliferation of life into so many environmental niches, so relatively soon after our planet became habitable, has served to make many scientists optimistic about the chances that life could exist elsewhere.
Glossary
- deuterium
- a form of hydrogen in which the nucleus of each atom consists of one proton and one neutron
- fusion
- the building of heavier atomic nuclei from lighter ones
- lithium
- the third element in the periodic table; lithium nuclei with three protons and four neutrons were manufactured during the first few minutes of the expansion of the universe
- cosmic microwave background (CMB)
- microwave radiation coming from all directions that is redshifted afterglow of the Big Bang
- flat universe
- a model of the universe that has a critical denisty and in which the geometry of the universe is flat, like a sheet of paper
- photon decoupling
- time when radiation began to stream freely through the universe without interacting with matter
- amino acids
- organic compounds that are the molecular building blocks of proteins
- astrobiology
- the multidisciplinary study of life in the universe: its origin, evolution, distribution, and fate; similar terms are exobiology and bioastronomy
- DNA (deoxyribonucleic acid)
- a molecule that stores information about how to replicate a cell and its chemical and structural components
- extremophile
- an organism (usually a microbe) that tolerates or even thrives under conditions that most of the life around us would consider hostile, such as very high or low temperature or acidity
- gene
- the basic functional unit that carries the genetic (hereditary) material contained in a cell
- habitable environment
- an environment capable of hosting life
- organic compound
- a compound containing carbon, especially a complex carbon compound; not necessarily produced by life
- photosynthesis
- a complex sequence of chemical reactions through which some living things can use sunlight to manufacture products that store energy (such as carbohydrates), releasing oxygen as one by-product
- protein
- a key biological molecule that provides the structure and function of the body’s tissues and organs, and essentially carries out the chemical work of the cell
- RNA (ribonucleic acid)
- a molecule that aids in the flow of genetic information from DNA to proteins
- stromatolites
- solid, layered rock formations that are thought to be the fossils of oxygen-producing photosynthetic bacteria in rocks that are 3.5 billion years old
- thermophile
- an organism that can tolerate high temperatures